Суперконденсатор
![]() | Эта статья может оказаться слишком длинной для удобного чтения и навигации . ( ноябрь 2023 г. ) |


Суперконденсатор которого намного выше , ( SC ), также называемый ультраконденсатором большой емкости , представляет собой конденсатор , значение емкости чем у твердотельных конденсаторов, но с более низкими пределами напряжения . Он заполняет пробел между электролитическими конденсаторами и аккумуляторными батареями . Обычно он хранит в 10–100 раз больше энергии на единицу объема или массы , чем электролитические конденсаторы, может принимать и доставлять заряд гораздо быстрее, чем батареи, и выдерживает гораздо больше циклов зарядки и разрядки, чем перезаряжаемые батареи. [2]
В отличие от обычных конденсаторов, в суперконденсаторах не используется обычный твердый диэлектрик , а используется электростатическая двухслойная емкость и электрохимическая псевдоемкость . [3] оба из которых способствуют накоплению общей энергии конденсатора.
Supercapacitors are used in applications requiring many rapid charge/discharge cycles, rather than long-term compact energy storage: in automobiles, buses, trains, cranes and elevators, where they are used for regenerative braking, short-term energy storage, or burst-mode power delivery.[4] Smaller units are used as power backup for static random-access memory (SRAM).
Background
[edit]The electrochemical charge storage mechanisms in solid media can be roughly (there is an overlap in some systems) classified into 3 types:
- Electrostatic double-layer capacitors (EDLCs) use carbon electrodes or derivatives with much higher electrostatic double-layer capacitance than electrochemical pseudocapacitance, achieving separation of charge in a Helmholtz double layer at the interface between the surface of a conductive electrode and an electrolyte. The separation of charge is of the order of a few ångströms (0.3–0.8 nm), much smaller than in a conventional capacitor. The electric charge in EDLCs is stored in a two-dimensional interphase (surface) of an electronic conductor (e.g. carbon particle) and ionic conductor (electrolyte solution).
- Batteries with solid electroactive materials store charge in bulk solid phases by virtue of redox chemical reactions.[5]
- Electrochemical supercapacitors (ECSCs) fall in between EDLs and batteries. ECSCs use metal oxide or conducting polymer electrodes with a high amount of electrochemical pseudocapacitance additional to the double-layer capacitance. Pseudocapacitance is achieved by Faradaic electron charge-transfer with redox reactions, intercalation or electrosorption.
In solid-state capacitors, the mobile charges are electrons, and the gap between electrodes is a layer of a dielectric. In electrochemical double-layer capacitors, the mobile charges are solvated ions (cations and anions), and the effective thickness is determined on each of the two electrodes by their electrochemical double layer structure. In batteries the charge is stored in the bulk volume of solid phases, which have both electronic and ionic conductivities. In electrochemical supercapacitors, the charge storage mechanisms either combine the double-layer and battery mechanisms, or are based on mechanisms, which are intermediate between true double layer and true battery.
History
[edit]
In the early 1950s, General Electric engineers began experimenting with porous carbon electrodes in the design of capacitors, from the design of fuel cells and rechargeable batteries. Activated charcoal is an electrical conductor that is an extremely porous "spongy" form of carbon with a high specific surface area. In 1957 H. Becker developed a "Low voltage electrolytic capacitor with porous carbon electrodes".[6][7][8] He believed that the energy was stored as a charge in the carbon pores as in the pores of the etched foils of electrolytic capacitors. Because the double layer mechanism was not known by him at the time, he wrote in the patent: "It is not known exactly what is taking place in the component if it is used for energy storage, but it leads to an extremely high capacity."
General Electric did not immediately pursue this work. In 1966 researchers at Standard Oil of Ohio (SOHIO) developed another version of the component as "electrical energy storage apparatus", while working on experimental fuel cell designs.[9][10] The nature of electrochemical energy storage was not described in this patent. Even in 1970, the electrochemical capacitor patented by Donald L. Boos was registered as an electrolytic capacitor with activated carbon electrodes.[11]
Early electrochemical capacitors used two aluminum foils covered with activated carbon (the electrodes) that were soaked in an electrolyte and separated by a thin porous insulator. This design gave a capacitor with a capacitance on the order of one farad, significantly higher than electrolytic capacitors of the same dimensions. This basic mechanical design remains the basis of most electrochemical capacitors.
SOHIO did not commercialize their invention, licensing the technology to NEC, who finally marketed the results as "supercapacitors" in 1978, to provide backup power for computer memory.[10]
Between 1975 and 1980 Brian Evans Conway conducted extensive fundamental and development work on ruthenium oxide electrochemical capacitors. In 1991 he described the difference between "supercapacitor" and "battery" behaviour in electrochemical energy storage. In 1999 he defined the term "supercapacitor" to make reference to the increase in observed capacitance by surface redox reactions with faradaic charge transfer between electrodes and ions.[12][13] His "supercapacitor" stored electrical charge partially in the Helmholtz double-layer and partially as result of faradaic reactions with "pseudocapacitance" charge transfer of electrons and protons between electrode and electrolyte. The working mechanisms of pseudocapacitors are redox reactions, intercalation and electrosorption (adsorption onto a surface). With his research, Conway greatly expanded the knowledge of electrochemical capacitors.
The market expanded slowly. That changed around 1978 as Panasonic marketed its Goldcaps brand.[14] This product became a successful energy source for memory backup applications.[10] Competition started only years later. In 1987 ELNA "Dynacap"s entered the market.[15] First generation EDLC's had relatively high internal resistance that limited the discharge current. They were used for low current applications such as powering SRAM chips or for data backup.
At the end of the 1980s, improved electrode materials increased capacitance values. At the same time, the development of electrolytes with better conductivity lowered the equivalent series resistance (ESR) increasing charge/discharge currents. The first supercapacitor with low internal resistance was developed in 1982 for military applications through the Pinnacle Research Institute (PRI), and were marketed under the brand name "PRI Ultracapacitor". In 1992, Maxwell Laboratories (later Maxwell Technologies) took over this development. Maxwell adopted the term Ultracapacitor from PRI and called them "Boost Caps"[16] to underline their use for power applications.
Since capacitors' energy content increases with the square of the voltage, researchers were looking for a way to increase the electrolyte's breakdown voltage. In 1994 using the anode of a 200 V high-voltage tantalum electrolytic capacitor, David A. Evans developed an "Electrolytic-Hybrid Electrochemical Capacitor".[17][18] These capacitors combine features of electrolytic and electrochemical capacitors. They combine the high dielectric strength of an anode from an electrolytic capacitor with the high capacitance of a pseudocapacitive metal oxide (ruthenium (IV) oxide) cathode from an electrochemical capacitor, yielding a hybrid electrochemical capacitor. Evans' capacitors, coined Capattery,[19] had an energy content about a factor of 5 higher than a comparable tantalum electrolytic capacitor of the same size.[20] Their high costs limited them to specific military applications.
Recent developments include lithium-ion capacitors. These hybrid capacitors were pioneered by Fujitsu's FDK in 2007.[21] They combine an electrostatic carbon electrode with a pre-doped lithium-ion electrochemical electrode. This combination increases the capacitance value. Additionally, the pre-doping process lowers the anode potential and results in a high cell output voltage, further increasing specific energy.
Research departments active in many companies and universities[22] are working to improve characteristics such as specific energy, specific power, and cycle stability and to reduce production costs.
Design
[edit]Basic design
[edit]Electrochemical capacitors (supercapacitors) consist of two electrodes separated by an ion-permeable membrane (separator), and an electrolyte ionically connecting both electrodes. When the electrodes are polarized by an applied voltage, ions in the electrolyte form electric double layers of opposite polarity to the electrode's polarity. For example, positively polarized electrodes will have a layer of negative ions at the electrode/electrolyte interface along with a charge-balancing layer of positive ions adsorbing onto the negative layer. The opposite is true for the negatively polarized electrode.
Additionally, depending on electrode material and surface shape, some ions may permeate the double layer becoming specifically adsorbed ions and contribute with pseudocapacitance to the total capacitance of the supercapacitor.
Capacitance distribution
[edit]The two electrodes form a series circuit of two individual capacitors C1 and C2. The total capacitance Ctotal is given by the formula
Supercapacitors may have either symmetric or asymmetric electrodes. Symmetry implies that both electrodes have the same capacitance value, yielding a total capacitance of half the value of each single electrode (if C1 = C2, then Ctotal = ½ C1). For asymmetric capacitors, the total capacitance can be taken as that of the electrode with the smaller capacitance (if C1 >> C2, then Ctotal ≈ C2).
Storage principles
[edit]Electrochemical capacitors use the double-layer effect to store electric energy; however, this double-layer has no conventional solid dielectric to separate the charges. There are two storage principles in the electric double-layer of the electrodes that contribute to the total capacitance of an electrochemical capacitor:[23]
- Double-layer capacitance, electrostatic storage of the electrical energy achieved by separation of charge in a Helmholtz double layer.[24]
- Pseudocapacitance, electrochemical storage of the electrical energy. The original type uses faradaic redox reactions with charge-transfer.[16]
Both capacitances are only separable by measurement techniques. The amount of charge stored per unit voltage in an electrochemical capacitor is primarily a function of the electrode size, although the amount of capacitance of each storage principle can vary extremely.
Electrical double-layer capacitance
[edit]
Every electrochemical capacitor has two electrodes, mechanically separated by a separator, which are ionically connected to each other via the electrolyte. The electrolyte is a mixture of positive and negative ions dissolved in a solvent such as water. At each of the two electrode surfaces originates an area in which the liquid electrolyte contacts the conductive metallic surface of the electrode. This interface forms a common boundary among two different phases of matter, such as an insoluble solid electrode surface and an adjacent liquid electrolyte. In this interface occurs a very special phenomenon of the double layer effect.[25]
Applying a voltage to an electrochemical capacitor causes both electrodes in the capacitor to generate electrical double-layers. These double-layers consist of two layers of charges: one electronic layer is in the surface lattice structure of the electrode, and the other, with opposite polarity, emerges from dissolved and solvated ions in the electrolyte. The two layers are separated by a monolayer of solvent molecules, e.g., for water as solvent by water molecules, called inner Helmholtz plane (IHP). Solvent molecules adhere by physical adsorption on the surface of the electrode and separate the oppositely polarized ions from each other, and can be idealised as a molecular dielectric. In the process, there is no transfer of charge between electrode and electrolyte, so the forces that cause the adhesion are not chemical bonds, but physical forces, e.g., electrostatic forces. The adsorbed molecules are polarized, but, due to the lack of transfer of charge between electrolyte and electrode, suffered no chemical changes.
The amount of charge in the electrode is matched by the magnitude of counter-charges in outer Helmholtz plane (OHP). This double-layer phenomena stores electrical charges as in a conventional capacitor. The double-layer charge forms a static electric field in the molecular layer of the solvent molecules in the IHP that corresponds to the strength of the applied voltage.

The double-layer serves approximately as the dielectric layer in a conventional capacitor, albeit with the thickness of a single molecule. Thus, the standard formula for conventional plate capacitors can be used to calculate their capacitance:[26]
- .
Accordingly, capacitance C is greatest in capacitors made from materials with a high permittivity ε, large electrode plate surface areas A and small distance between plates d. As a result, double-layer capacitors have much higher capacitance values than conventional capacitors, arising from the extremely large surface area of activated carbon electrodes and the extremely thin double-layer distance on the order of a few ångströms (0.3-0.8 nm), of order of the Debye length.[16][24]
Assuming that the minimum distance between the electrode and the charge accumulating region cannot be less than the typical distance between negative and positive charges in atoms of ~0.05 nm a general capacitance upper limit of ~18 μF/cm2 has been predicted for non-faradaic capacitors.[27]
The main drawback of carbon electrodes of double-layer SCs is small values of quantum capacitance[citation needed] which act in series[28] with capacitance of ionic space charge. Therefore, further increase of density of capacitance in SCs can be connected with increasing of quantum capacitance of carbon electrode nanostructures.[citation needed]
The amount of charge stored per unit voltage in an electrochemical capacitor is primarily a function of the electrode size. The electrostatic storage of energy in the double-layers is linear with respect to the stored charge, and correspond to the concentration of the adsorbed ions. Also, while charge in conventional capacitors is transferred via electrons, capacitance in double-layer capacitors is related to the limited moving speed of ions in the electrolyte and the resistive porous structure of the electrodes. Since no chemical changes take place within the electrode or electrolyte, charging and discharging electric double-layers in principle is unlimited. Real supercapacitors lifetimes are only limited by electrolyte evaporation effects.
Electrochemical pseudocapacitance
[edit]
Applying a voltage at the electrochemical capacitor terminals moves electrolyte ions to the opposite polarized electrode and forms a double-layer in which a single layer of solvent molecules acts as separator. Pseudocapacitance can originate when specifically adsorbed ions out of the electrolyte pervade the double-layer. This pseudocapacitance stores electrical energy by means of reversible faradaic redox reactions on the surface of suitable electrodes in an electrochemical capacitor with an electric double-layer.[12][23][24][29][30] Pseudocapacitance is accompanied with an electron charge-transfer between electrolyte and electrode coming from a de-solvated and adsorbed ion whereby only one electron per charge unit is participating. This faradaic charge transfer originates by a very fast sequence of reversible redox, intercalation or electrosorption processes. The adsorbed ion has no chemical reaction with the atoms of the electrode (no chemical bonds arise[31]) since only a charge-transfer take place.

The electrons involved in the faradaic processes are transferred to or from valence electron states (orbitals) of the redox electrode reagent. They enter the negative electrode and flow through the external circuit to the positive electrode where a second double-layer with an equal number of anions has formed. The electrons reaching the positive electrode are not transferred to the anions forming the double-layer, instead they remain in the strongly ionized and "electron hungry" transition-metal ions of the electrode's surface. As such, the storage capacity of faradaic pseudocapacitance is limited by the finite quantity of reagent in the available surface.
A faradaic pseudocapacitance only occurs together with a static double-layer capacitance, and its magnitude may exceed the value of double-layer capacitance for the same surface area by factor of 100, depending on the nature and the structure of the electrode, because all the pseudocapacitance reactions take place only with de-solvated ions, which are much smaller than solvated ion with their solvating shell.[12][29] The amount of pseudocapacitance has a linear function within narrow limits determined by the potential-dependent degree of surface coverage of the adsorbed anions.
The ability of electrodes to accomplish pseudocapacitance effects by redox reactions, intercalation or electrosorption strongly depends on the chemical affinity of electrode materials to the ions adsorbed on the electrode surface as well as on the structure and dimension of the electrode pores. Materials exhibiting redox behavior for use as electrodes in pseudocapacitors are transition-metal oxides like RuO2, IrO2, or MnO2 inserted by doping in the conductive electrode material such as active carbon, as well as conducting polymers such as polyaniline or derivatives of polythiophene covering the electrode material.
The amount of electric charge stored in a pseudocapacitance is linearly proportional to the applied voltage. The unit of pseudocapacitance is farad, same as that of capacitance.
Although conventional battery-type electrode materials also use chemical reactions to store charge, they show very different electrical profiles, as the rate of discharge is limited by the speed of diffusion. Grinding those materials down to nanoscale frees them of the diffusion limit and give them a more pseudocapacitative behavior, making them extrinsic pseudocapacitors. Chodankar et al. 2020, figure 2 shows the representative voltage-capacity curves for bulk LiCoO2, nano LiCoO2, a redox pseudocapacitor (RuO2), and a intercalation pseudocapacitor (T-Nb2O5).[32]: 5
Asymmetric capacitors
[edit]Supercapacitors can also be made with different materials and principles at the electrodes. If both of those materials use a fast, supercapacitor-type reaction (capacitance or pseudocapacitance), the result is called an asymmetric capacitor. The two electrodes have different electric potentials; when combined with proper balancing, the result is improved energy density with no loss of lifespan or current capacity.[32]: 8
Hybrid capacitors
[edit]A number of newer supercapacitors are "hybrid": only one electrode uses a fast reaction (capacitance or pseudocapacitance), the other using a more "battery-like" (slower but higher-capacity) material. For example, an EDLC anode can be combined with an activated carbon–Ni(OH)2 cathode, the latter being a slow faradaic material. The CV and GCD profiles of a hybrid capacitor have a shape between that of a battery and an SC, more similar to that of an SC. Hybrid capacitors have much higher energy density, but have inferior cycle life and current capacity owing to the slower electrode.[32]: 7
Potential distribution
[edit]


Conventional capacitors (also known as electrostatic capacitors), such as ceramic capacitors and film capacitors, consist of two electrodes separated by a dielectric material. When charged, the energy is stored in a static electric field that permeates the dielectric between the electrodes. The total energy increases with the amount of stored charge, which in turn correlates linearly with the potential (voltage) between the plates. The maximum potential difference between the plates (the maximal voltage) is limited by the dielectric's breakdown field strength. The same static storage also applies for electrolytic capacitors in which most of the potential decreases over the anode's thin oxide layer. The somewhat resistive liquid electrolyte (cathode) accounts for a small decrease of potential for "wet" electrolytic capacitors, while electrolytic capacitors with solid conductive polymer electrolyte this voltage drop is negligible.
In contrast, electrochemical capacitors (supercapacitors) consists of two electrodes separated by an ion-permeable membrane (separator) and electrically connected via an electrolyte. Energy storage occurs within the double-layers of both electrodes as a mixture of a double-layer capacitance and pseudocapacitance. When both electrodes have approximately the same resistance (internal resistance), the potential of the capacitor decreases symmetrically over both double-layers, whereby a voltage drop across the equivalent series resistance (ESR) of the electrolyte is achieved. For asymmetrical supercapacitors like hybrid capacitors the voltage drop between the electrodes could be asymmetrical. The maximum potential across the capacitor (the maximal voltage) is limited by the electrolyte decomposition voltage.
Both electrostatic and electrochemical energy storage in supercapacitors are linear with respect to the stored charge, just as in conventional capacitors. The voltage between the capacitor terminals is linear with respect to the amount of stored energy. Such linear voltage gradient differs from rechargeable electrochemical batteries, in which the voltage between the terminals remains independent of the amount of stored energy, providing a relatively constant voltage.
Comparison with other storage technologies
[edit]Supercapacitors compete with electrolytic capacitors and rechargeable batteries, especially lithium-ion batteries. The following table compares the major parameters of the three main supercapacitor families with electrolytic capacitors and batteries.
Parameter | Aluminum electrolytic capacitors | Supercapacitors | Lithium-ion batteries | |||
---|---|---|---|---|---|---|
Double-layer capacitors (memory backup) | Pseudocapacitors | Hybrid (Li-ion) | NTGS EDLC[33] (experimental) | |||
Temperature range, degrees Celsius (°C) | −40 – +125 °C | −40 – +70 °C | −20 – +70 °C | −20 – +70 °C | −20 – +60 °C | |
Maximum charge, volts (V) | 4 – 630 V | 1.2 – 3.3 V | 2.2 – 3.3 V | 2.2 – 3.8 V | ~ 4.0 V | 2.5 – 4.2 V |
Recharge cycles, thousands (k) | < unlimited | 100 k – 1 000 k | 100 k – 1 000 k | 20 k – 100 k | > 20 k | 0.5 k – 10 k |
Capacitance, farads (F) | ≤ 2.7 F | 0.1 – 470 F | 100 – 12 000 F | 300 – 3 300 F | — | |
Specific energy, watt-hours per kilogram (Wh/kg) | 0.01 – 0.3 Wh/kg | 1.5 – 3.9 Wh/kg | 4 – 9 Wh/kg | 10 – 15 Wh/kg | 206 Wh/kg | 100 – 265 Wh/kg |
Specific power, watts per gram (W/g) | > 100 W/g | 2 – 10 W/g | 3 – 10 W/g | 3 – 14 W/g | 32 W/g | 0.3 – 1.5 W/g |
Self-discharge time at room temp. | short (days) | medium (weeks) | medium (weeks) | long (month) | long (month) | |
Efficiency (%) | 99% | 95% | 95% | 90% | 90% | |
Working life at room temp., years (y) | > 20 y | 5 – 10 y | 5 – 10 y | 5 – 10 y | 3 – 5 y |
Electrolytic capacitors feature nearly unlimited charge/discharge cycles, high dielectric strength (up to 550 V) and good frequency response as alternating current (AC) reactance in the lower frequency range. Supercapacitors can store 10 to 100 times more energy than electrolytic capacitors, but they do not support AC applications.
With regards to rechargeable batteries, supercapacitors feature higher peak currents, low cost per cycle, no danger of overcharging, good reversibility, non-corrosive electrolyte and low material toxicity. Batteries offer lower purchase cost and stable voltage under discharge, but require complex electronic control and switching equipment, with consequent energy loss and spark hazard given a short.[clarification needed]
Styles
[edit]
1. terminals, 2. safety vent, 3. sealing disc, 4. aluminum can, 5. positive pole, 6. separator, 7. carbon electrode, 8. collector, 9. carbon electrode, 10. negative pole
1. positive electrode, 2. negative electrode, 3. separator
Supercapacitors are made in different styles, such as flat with a single pair of electrodes, wound in a cylindrical case, or stacked in a rectangular case. Because they cover a broad range of capacitance values, the size of the cases can vary.
Supercapacitors are constructed with two metal foils (current collectors), each coated with an electrode material such as activated carbon, which serve as the power connection between the electrode material and the external terminals of the capacitor. Specifically to the electrode material is a very large surface area. In this example the activated carbon is electrochemically etched, so that the surface area of the material is about 100,000 times greater than the smooth surface. The electrodes are kept apart by an ion-permeable membrane (separator) used as an insulator to protect the electrodes against short circuits. This construction is subsequently rolled or folded into a cylindrical or rectangular shape and can be stacked in an aluminum can or an adaptable rectangular housing. The cell is then impregnated with a liquid or viscous electrolyte of organic or aqueous type. The electrolyte, an ionic conductor, enters the pores of the electrodes and serves as the conductive connection between the electrodes across the separator. Finally, the housing is hermetically sealed to ensure stable behavior over the specified lifetime.
Types
[edit]
Electrical energy is stored in supercapacitors via two storage principles, static double-layer capacitance and electrochemical pseudocapacitance; and the distribution of the two types of capacitance depends on the material and structure of the electrodes. There are three types of supercapacitors based on storage principle:[16][24]
- Double-layer capacitors (EDLCs): with activated carbon electrodes or derivatives with much higher electrostatic double-layer capacitance than electrochemical pseudocapacitance
- Pseudocapacitors: with transition metal oxide or conducting polymer electrodes with a high electrochemical pseudocapacitance
- Hybrid capacitors: with asymmetric electrodes, one of which exhibits mostly electrostatic and the other mostly electrochemical capacitance, such as lithium-ion capacitors
Because double-layer capacitance and pseudocapacitance both contribute inseparably to the total capacitance value of an electrochemical capacitor, a correct description of these capacitors only can be given under the generic term. The concepts of supercapattery and supercabattery have been recently proposed to better represent those hybrid devices that behave more like the supercapacitor and the rechargeable battery, respectively.[34]
The capacitance value of a supercapacitor is determined by two storage principles:
- Double-layer capacitance – electrostatic storage of the electrical energy achieved by separation of charge in a Helmholtz double layer at the interface between the surface of a conductor electrode and an electrolytic solution electrolyte. The separation of charge distance in a double-layer is on the order of a few ångströms (0.3–0.8 nm) and is static in origin.[16]
- Pseudocapacitance – Electrochemical storage of the electrical energy, achieved by redox reactions, electrosorption or intercalation on the surface of the electrode by specifically adsorbed ions, that results in a reversible faradaic charge-transfer on the electrode.[16]
Double-layer capacitance and pseudocapacitance both contribute inseparably to the total capacitance value of a supercapacitor.[23] However, the ratio of the two can vary greatly, depending on the design of the electrodes and the composition of the electrolyte. Pseudocapacitance can increase the capacitance value by as much as a factor of ten over that of the double-layer by itself.[12][29]
Electric double-layer capacitors (EDLC) are electrochemical capacitors in which energy storage predominantly is achieved by double-layer capacitance. In the past, all electrochemical capacitors were called "double-layer capacitors". Contemporary usage sees double-layer capacitors, together with pseudocapacitors, as part of a larger family of electrochemical capacitors[12][29] called supercapacitors. They are also known as ultracapacitors.
Materials
[edit]The properties of supercapacitors come from the interaction of their internal materials. Especially, the combination of electrode material and type of electrolyte determine the functionality and thermal and electrical characteristics of the capacitors.
Electrodes
[edit]
Supercapacitor electrodes are generally thin coatings applied and electrically connected to a conductive, metallic current collector. Electrodes must have good conductivity, high temperature stability, long-term chemical stability (inertness), high corrosion resistance and high surface areas per unit volume and mass. Other requirements include environmental friendliness and low cost.
The amount of double-layer as well as pseudocapacitance stored per unit voltage in a supercapacitor is predominantly a function of the electrode surface area. Therefore, supercapacitor electrodes are typically made of porous, spongy material with an extraordinarily high specific surface area, such as activated carbon. Additionally, the ability of the electrode material to perform faradaic charge transfers enhances the total capacitance.
Generally the smaller the electrode's pores, the greater the capacitance and specific energy. However, smaller pores increase equivalent series resistance (ESR) and decrease specific power. Applications with high peak currents require larger pores and low internal losses, while applications requiring high specific energy need small pores.
Electrodes for EDLCs
[edit]The most commonly used electrode material for supercapacitors is carbon in various manifestations such as activated carbon (AC), carbon fibre-cloth (AFC), carbide-derived carbon (CDC),[35][36] carbon aerogel, graphite (graphene), graphane[37] and carbon nanotubes (CNTs).[23][38][39]
Carbon-based electrodes exhibit predominantly static double-layer capacitance, even though a small amount of pseudocapacitance may also be present depending on the pore size distribution. Pore sizes in carbons typically range from micropores (less than 2 nm) to mesopores (2-50 nm),[40] but only micropores (<2 nm) contribute to pseudocapacitance. As pore size approaches the solvation shell size, solvent molecules are excluded and only unsolvated ions fill the pores (even for large ions), increasing ionic packing density and storage capability by faradaic H
2 intercalation.[23]
Activated carbon
[edit]Activated carbon was the first material chosen for EDLC electrodes. Even though its electrical conductivity is approximately 0.003% that of metals (1,250 to 2,000 S/m), it is sufficient for supercapacitors.[24][16] Activated carbon is an extremely porous form of carbon with a high specific surface area — a common approximation is that 1 gram (0.035 oz) (a pencil-eraser-sized amount) has a surface area of roughly 1,000 to 3,000 square metres (11,000 to 32,000 sq ft)[38][40] — about the size of 4 to 12 tennis courts. The bulk form used in electrodes is low-density with many pores, giving high double-layer capacitance. Solid activated carbon, also termed consolidated amorphous carbon (CAC) is the most used electrode material for supercapacitors and may be cheaper than other carbon derivatives.[41] It is produced from activated carbon powder pressed into the desired shape, forming a block with a wide distribution of pore sizes. An electrode with a surface area of about 1000 m2/g results in a typical double-layer capacitance of about 10 μF/cm2 and a specific capacitance of 100 F/g. As of 2010[update] virtually all commercial supercapacitors use powdered activated carbon made from coconut shells.[42] Coconut shells produce activated carbon with more micropores than does charcoal made from wood.[40]
Activated carbon fibres
[edit]Activated carbon fibres (ACF) are produced from activated carbon and have a typical diameter of 10 μm. They can have micropores with a very narrow pore-size distribution that can be readily controlled. The surface area of ACF woven into a textile is about 2500 m2/g. Advantages of ACF electrodes include low electrical resistance along the fibre axis and good contact to the collector.[38] As for activated carbon, ACF electrodes exhibit predominantly double-layer capacitance with a small amount of pseudocapacitance due to their micropores.
Carbon aerogel
[edit]
Carbon aerogel is a highly porous, synthetic, ultralight material derived from an organic gel in which the liquid component of the gel has been replaced with a gas. Aerogel electrodes are made via pyrolysis of resorcinol-formaldehyde aerogels[43] and are more conductive than most activated carbons. They enable thin and mechanically stable electrodes with a thickness in the range of several hundred micrometres (μm) and with uniform pore size. Aerogel electrodes also provide mechanical and vibration stability for supercapacitors used in high-vibration environments. Researchers have created a carbon aerogel electrode with gravimetric densities of about 400–1200 m2/g and volumetric capacitance of 104 F/cm3, yielding a specific energy of 325 kJ/kg (90 Wh/kg) and specific power of 20 W/g.[44][45] Standard aerogel electrodes exhibit predominantly double-layer capacitance. Aerogel electrodes that incorporate composite material can add a high amount of pseudocapacitance.[46]
Carbide-derived carbon
[edit]
Carbide-derived carbon (CDC), also known as tunable nanoporous carbon, is a family of carbon materials derived from carbide precursors, such as binary silicon carbide and titanium carbide, that are transformed into pure carbon via physical, e.g., thermal decomposition or chemical, e.g., halogenation) processes.[47][48] Carbide-derived carbons can exhibit high surface area and tunable pore diameters (from micropores to mesopores) to maximize ion confinement, increasing pseudocapacitance by faradaic H
2 adsorption treatment. CDC electrodes with tailored pore design offer as much as 75% greater specific energy than conventional activated carbons. As of 2015[update], a CDC supercapacitor offered a specific energy of 10.1 Wh/kg, 3,500 F capacitance and over one million charge-discharge cycles.[49]
Graphene
[edit]
Graphene is a one-atom thick sheet of graphite, with atoms arranged in a regular hexagonal pattern,[50][51] also called "nanocomposite paper".[52]
Graphene has a theoretical specific surface area of 2630 m2/g which can theoretically lead to a capacitance of 550 F/g. In addition, an advantage of graphene over activated carbon is its higher electrical conductivity. As of 2012[update] a new development used graphene sheets directly as electrodes without collectors for portable applications.[53][54]
In one embodiment, a graphene-based supercapacitor uses curved graphene sheets that do not stack face-to-face, forming mesopores that are accessible to and wettable by ionic electrolytes at voltages up to 4 V. A specific energy of 85.6 Wh/kg (308 kJ/kg) is obtained at room temperature equaling that of a conventional nickel metal hydride battery, but with 100-1000 times greater specific power.[55][56]
The two-dimensional structure of graphene improves charging and discharging. Charge carriers in vertically oriented sheets can quickly migrate into or out of the deeper structures of the electrode, thus increasing currents. Such capacitors may be suitable for 100/120 Hz filter applications, which are unreachable for supercapacitors using other carbon materials.[57]
Carbon nanotubes
[edit]
Carbon nanotubes (CNTs), also called buckytubes, are carbon molecules with a cylindrical nanostructure. They have a hollow structure with walls formed by one-atom-thick sheets of graphite. These sheets are rolled at specific and discrete ("chiral") angles, and the combination of chiral angle and radius controls properties such as electrical conductivity, electrolyte wettability and ion access. Nanotubes are categorized as single-walled nanotubes (SWNTs) or multi-walled nanotubes (MWNTs). The latter have one or more outer tubes successively enveloping a SWNT, much like the Russian matryoshka dolls. SWNTs have diameters ranging between 1 and 3 nm. MWNTs have thicker coaxial walls, separated by spacing (0.34 nm) that is close to graphene's interlayer distance.
Nanotubes can grow vertically on the collector substrate, such as a silicon wafer. Typical lengths are 20 to 100 μm.[58]
Carbon nanotubes can greatly improve capacitor performance, due to the highly wettable surface area and high conductivity.[59][60]
A SWNT-based supercapacitor with aqueous electrolyte was systematically studied at University of Delaware in Prof. Bingqing Wei's group. Li et al., for the first time, discovered that the ion-size effect and the electrode-electrolyte wettability are the dominant factors affecting the electrochemical behavior of flexible SWCNTs-supercapacitors in different 1 molar aqueous electrolytes with different anions and cations. The experimental results also showed for flexible supercapacitor that it is suggested to put enough pressure between the two electrodes to improve the aqueous electrolyte CNT supercapacitor.[61]
CNTs can store about the same charge as activated carbon per unit surface area, but nanotubes' surface is arranged in a regular pattern, providing greater wettability. SWNTs have a high theoretical specific surface area of 1315 m2/g, while that for MWNTs is lower and is determined by the diameter of the tubes and degree of nesting, compared with a surface area of about 3000 m2/g of activated carbons. Nevertheless, CNTs have higher capacitance than activated carbon electrodes, e.g., 102 F/g for MWNTs and 180 F/g for SWNTs.[citation needed]
MWNTs have mesopores that allow for easy access of ions at the electrode–electrolyte interface. As the pore size approaches the size of the ion solvation shell, the solvent molecules are partially stripped, resulting in larger ionic packing density and increased faradaic storage capability. However, the considerable volume change during repeated intercalation and depletion decreases their mechanical stability. To this end, research to increase surface area, mechanical strength, electrical conductivity and chemical stability is ongoing.[59][62][63]
Electrodes for pseudocapacitors
[edit]MnO2 and RuO2 are typical materials used as electrodes for pseudocapacitors, since they have the electrochemical signature of a capacitive electrode (linear dependence on current versus voltage curve) as well as exhibiting aic behavior. Additionally, the charge storage originates from electron-transfer mechanisms rather than accumulation of ions in the electrochemical double layer. Pseudocapacitors were created through faradaic redox reactions that occur within the active electrode materials. More research was focused on transition-metal oxides such as MnO2 since transition-metal oxides have a lower cost compared to noble metal oxides such as RuO2. Moreover, the charge storage mechanisms of transition-metal oxides are based predominantly on pseudocapacitance. Two mechanisms of MnO2 charge storage behavior were introduced. The first mechanism implies the intercalation of protons (H+) or alkali metal cations (C+) in the bulk of the material upon reduction followed by deintercalation upon oxidation.[64]
- MnO2 + H+ (C+) + e− ⇌ MnOOH(C)[65]
The second mechanism is based on the surface adsorption of electrolyte cations on MnO2.
- (MnO2)surface + C+ + e− ⇌ (MnO2− C+)surface
Not every material that exhibits faradaic behavior can be used as an electrode for pseudocapacitors, such as Ni(OH)2 since it is a battery type electrode (non-linear dependence on current versus voltage curve).[66]
Metal oxides
[edit]Brian Evans Conway's research[12][13] described electrodes of transition metal oxides that exhibited high amounts of pseudocapacitance. Oxides of transition metals including ruthenium (RuO
2), iridium (IrO
2), iron (Fe
3O
4), manganese (MnO
2) or sulfides such as titanium sulfide (TiS
2) alone or in combination generate strong faradaic electron–transferring reactions combined with low resistance.[citation needed] Ruthenium dioxide in combination with H
2SO
4 electrolyte provides specific capacitance of 720 F/g and a high specific energy of 26.7 Wh/kg (96.12 kJ/kg).[67]
Charge/discharge takes place over a window of about 1.2 V per electrode. This pseudocapacitance of about 720 F/g is roughly 100 times higher than for double-layer capacitance using activated carbon electrodes. These transition metal electrodes offer excellent reversibility, with several hundred-thousand cycles. However, ruthenium is expensive and the 2.4 V voltage window for this capacitor limits their applications to military and space applications. Das et al. reported highest capacitance value (1715 F/g) for ruthenium oxide based supercapacitor with electrodeposited ruthenium oxide onto porous single wall carbon nanotube film electrode.[68] A high specific capacitance of 1715 F/g has been reported which closely approaches the predicted theoretical maximum RuO
2 capacitance of 2000 F/g.
In 2014, a RuO
2 supercapacitor anchored on a graphene foam electrode delivered specific capacitance of 502.78 F/g and areal capacitance of 1.11 F/cm2) leading to a specific energy of 39.28 Wh/kg and specific power of 128.01 kW/kg over 8,000 cycles with constant performance. The device was a three-dimensional (3D) sub-5 nm hydrous ruthenium-anchored graphene and carbon nanotube (CNT) hybrid foam (RGM) architecture. The graphene foam was conformally covered with hybrid networks of RuO
2 nanoparticles and anchored CNTs.[69][70]
Less expensive oxides of iron, vanadium, nickel and cobalt have been tested in aqueous electrolytes, but none has been investigated as much as manganese dioxide (MnO
2). However, none of these oxides are in commercial use.[71]
Conductive polymers
[edit]Another approach uses electron-conducting polymers as pseudocapacitive material. Although mechanically weak, conductive polymers have high conductivity, resulting in a low ESR[clarification needed] and a relatively high capacitance. Such conducting polymers include polyaniline, polythiophene, polypyrrole and polyacetylene. Such electrodes also employ electrochemical doping or dedoping of the polymers with anions and cations. Electrodes made from, or coated with, conductive polymers have costs comparable to carbon electrodes.
Conducting polymer electrodes generally suffer from limited cycling stability.[citation needed] However, polyacene electrodes provide up to 10,000 cycles, much better than batteries.[72]
Electrodes for hybrid capacitors
[edit]All commercial hybrid supercapacitors are asymmetric. They combine an electrode with high amount of pseudocapacitance with an electrode with a high amount of double-layer capacitance. In such systems the faradaic pseudocapacitance electrode with their higher capacitance provides high specific energy while the non-faradaic EDLC electrode enables high specific power. An advantage of the hybrid-type supercapacitors compared with symmetrical EDLC's is their higher specific capacitance value as well as their higher rated voltage and correspondingly their higher specific energy.[citation needed]
Composite electrodes
[edit]Composite electrodes for hybrid-type supercapacitors are constructed from carbon-based material with incorporated or deposited pseudocapacitive active materials like metal oxides and conducting polymers. As of 2013[update] most research for supercapacitors explores composite electrodes. CNTs give a backbone for a homogeneous distribution of metal oxide or electrically conducting polymers (ECPs), producing good pseudocapacitance and good double-layer capacitance. These electrodes achieve higher capacitances than either pure carbon or pure metal oxide or polymer-based electrodes. This is attributed to the accessibility of the nanotubes' tangled mat structure, which allows a uniform coating of pseudocapacitive materials and three-dimensional charge distribution. The process to anchor pseudocapactive materials usually uses a hydrothermal process. However, a recent researcher, Li et al., from the University of Delaware found a facile and scalable approach to precipitate MnO2 on a SWNT film to make an organic-electrolyte based supercapacitor.[73]
Another way to enhance CNT electrodes is by doping with a pseudocapacitive dopant as in lithium-ion capacitors. In this case the relatively small lithium atoms intercalate between the layers of carbon.[74] The anode is made of lithium-doped carbon, which enables lower negative potential with a cathode made of activated carbon. This results in a larger voltage of 3.8-4 V that prevents electrolyte oxidation. As of 2007 they had achieved capacitance of 550 F/g.[10] and reach a specific energy up to 14 Wh/kg (50.4 kJ/kg).[75]
Battery-type electrodes
[edit]Rechargeable battery electrodes influenced the development of electrodes for new hybrid-type supercapacitor electrodes as for lithium-ion capacitors.[76] Together with a carbon EDLC electrode in an asymmetric construction offers this configuration higher specific energy than typical supercapacitors with higher specific power, longer cycle life and faster charging and recharging times than batteries.
Asymmetric electrodes (pseudo/EDLC)
[edit]Recently some asymmetric hybrid supercapacitors were developed in which the positive electrode were based on a real pseudocapacitive metal oxide electrode (not a composite electrode), and the negative electrode on an EDLC activated carbon electrode.
Asymmetric supercapacitors (ASC) have shown a great potential candidate for high-performance supercapacitor due to their wide operating potential which can remarkably enhance the capacitive behavior. An advantage of this type of supercapacitors is their higher voltage and correspondingly their higher specific energy (up to 10-20 Wh/kg (36-72 kJ/kg)).[citation needed]And they also have good cycling stability.[77][78][79][80]
For example, researchers use a kind of novel skutterudite Ni–CoP3 nanosheets and use it as positive electrodes with activated carbon (AC) as negative electrodes to fabricate asymmetric supercapacitor (ASC). It exhibits high energy density of 89.6 Wh/kg at 796 W/kg and stability of 93% after 10,000 cycles, which can be a great potential to be an excellent next-generation electrode candidate.[80] Also, carbon nanofibers/poly(3,4-ethylenedioxythiophene)/manganese oxide (f-CNFs/PEDOT/MnO2) were used as positive electrodes and AC as negative electrodes. It has high specific energy of 49.4 Wh/kg and good cycling stability (81.06% after cycling 8000 times).[78] Besides, many kinds of nanocomposite are being studied as electrodes, like NiCo2S4@NiO,[79] MgCo2O4@MnO2 and so on. For example, Fe-SnO2@CeO2 nanocomposite used as electrode can provide a specific energy and specific power of 32.2 Wh/kg and 747 W/kg. The device exhibited the capacitance retention of 85.05 % over 5000 cycles of operation.[77] As far as known no commercial offered supercapacitors with such kind of asymmetric electrodes are on the market.
Electrolytes
[edit]Electrolytes consist of a solvent and dissolved chemicals that dissociate into positive cations and negative anions, making the electrolyte electrically conductive. The more ions the electrolyte contains, the better its conductivity. In supercapacitors electrolytes are the electrically conductive connection between the two electrodes. Additionally, in supercapacitors the electrolyte provides the molecules for the separating monolayer in the Helmholtz double-layer and delivers the ions for pseudocapacitance.
The electrolyte determines the capacitor's characteristics: its operating voltage, temperature range, ESR and capacitance. With the same activated carbon electrode an aqueous electrolyte achieves capacitance values of 160 F/g, while an organic electrolyte achieves only 100 F/g.[81]
The electrolyte must be chemically inert and not chemically attack the other materials in the capacitor to ensure long time stable behavior of the capacitor's electrical parameters. The electrolyte's viscosity must be low enough to wet the porous, sponge-like structure of the electrodes. An ideal electrolyte does not exist, forcing a compromise between performance and other requirements.
Water is a relatively good solvent for inorganic chemicals. Treated with acids such as sulfuric acid (H
2SO
4), alkalis such as potassium hydroxide (KOH), or salts such as quaternary phosphonium salts, sodium perchlorate (NaClO
4), lithium perchlorate (LiClO
4) or lithium hexafluoride arsenate (LiAsF
6), water offers relatively high conductivity values of about 100 to 1000 mS/cm. Aqueous electrolytes have a dissociation voltage of 1.15 V per electrode (2.3 V capacitor voltage) and a relatively low operating temperature range. They are used in supercapacitors with low specific energy and high specific power.
Electrolytes with organic solvents such as acetonitrile, propylene carbonate, tetrahydrofuran, diethyl carbonate, γ-butyrolactone and solutions with quaternary ammonium salts or alkyl ammonium salts such as tetraethylammonium tetrafluoroborate (N(Et)
4BF
4[82]) or triethyl (metyl) tetrafluoroborate (NMe(Et)
3BF
4) are more expensive than aqueous electrolytes, but they have a higher dissociation voltage of typically 1.35 V per electrode (2.7 V capacitor voltage), and a higher temperature range. The lower electrical conductivity of organic solvents (10 to 60 mS/cm) leads to a lower specific power, but since the specific energy increases with the square of the voltage, a higher specific energy.
Ionic electrolytes consists of liquid salts that can be stable in a wider electrochemical window, enabling capacitor voltages above 3.5 V. Ionic electrolytes typically have an ionic conductivity of a few mS/cm, lower than aqueous or organic electrolytes.[83]
Separators
[edit]Separators have to physically separate the two electrodes to prevent a short circuit by direct contact. It can be very thin (a few hundredths of a millimeter) and must be very porous to the conducting ions to minimize ESR. Furthermore, separators must be chemically inert to protect the electrolyte's stability and conductivity. Inexpensive components use open capacitor papers. More sophisticated designs use nonwoven porous polymeric films like polyacrylonitrile or Kapton, woven glass fibers or porous woven ceramic fibres.[84][85]
Collectors and housing
[edit]Current collectors connect the electrodes to the capacitor's terminals. The collector is either sprayed onto the electrode or is a metal foil. They must be able to distribute peak currents of up to 100 A. If the housing is made out of a metal (typically aluminum) the collectors should be made from the same material to avoid forming a corrosive galvanic cell.
Electrical parameters
[edit]Capacitance
[edit]


Capacitance values for commercial capacitors are specified as "rated capacitance CR". This is the value for which the capacitor has been designed. The value for an actual component must be within the limits given by the specified tolerance. Typical values are in the range of farads (F), three to six orders of magnitude larger than those of electrolytic capacitors. The capacitance value results from the energy (expressed in Joule) of a loaded capacitor loaded via a DC voltage VDC.
This value is also called the "DC capacitance".
Measurement
[edit]
Conventional capacitors are normally measured with a small AC voltage (0.5 V) and a frequency of 100 Hz or 1 kHz depending on the capacitor type. The AC capacitance measurement offers fast results, important for industrial production lines. The capacitance value of a supercapacitor depends strongly on the measurement frequency, which is related to the porous electrode structure and the limited electrolyte's ion mobility. Even at a low frequency of 10 Hz, the measured capacitance value drops from 100 to 20 percent of the DC capacitance value.
This extraordinarily strong frequency dependence can be explained by the different distances the ions have to move in the electrode's pores. The area at the beginning of the pores can be easily accessed by the ions; this short distance is accompanied by low electrical resistance. The greater the distance the ions have to cover, the higher the resistance. This phenomenon can be described with a series circuit of cascaded RC (resistor/capacitor) elements with serial RC time constants. These result in delayed current flow, reducing the total electrode surface area that can be covered with ions if polarity changes – capacitance decreases with increasing AC frequency. Thus, the total capacitance is achieved only after longer measuring times. Out of the reason of the very strong frequency dependence of the capacitance, this electrical parameter has to be measured with a special constant current charge and discharge measurement, defined in IEC standards 62391-1 and -2.
Measurement starts with charging the capacitor. The voltage has to be applied and after the constant current/constant voltage power supply has achieved the rated voltage, the capacitor must be charged for 30 minutes. Next, the capacitor has to be discharged with a constant discharge current Idischarge. Then the time t1 and t2, for the voltage to drop from 80% (V1) to 40% (V2) of the rated voltage is measured. The capacitance value is calculated as:
The value of the discharge current is determined by the application. The IEC standard defines four classes:
- Memory backup, discharge current in mA = 1 • C (F)
- Energy storage, discharge current in mA = 0,4 • C (F) • V (V)
- Power, discharge current in mA = 4 • C (F) • V (V)
- Instantaneous power, discharge current in mA = 40 • C (F) • V (V)
The measurement methods employed by individual manufacturers are mainly comparable to the standardized methods.[86][87]
The standardized measuring method is too time consuming for manufacturers to use during production for each individual component. For industrial-produced capacitors, the capacitance value is instead measured with a faster, low-frequency AC voltage, and a correlation factor is used to compute the rated capacitance.
This frequency dependence affects capacitor operation. Rapid charge and discharge cycles mean that neither the rated capacitance value nor specific energy are available. In this case the rated capacitance value is recalculated for each application condition.
The time t a supercapacitor can deliver a constant current I can be calculated as:
as the capacitor voltage decreases from Ucharge down to Umin.
If the application needs a constant power P for a certain time t this can be calculated as:
wherein also the capacitor voltage decreases from Ucharge down to Umin.
Operating voltage
[edit]
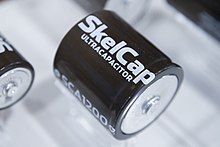
Supercapacitors are low voltage components. Safe operation requires that the voltage remain within specified limits. The rated voltage UR is the maximum DC voltage or peak pulse voltage that may be applied continuously and remain within the specified temperature range. Capacitors should never be subjected to voltages continuously in excess of the rated voltage.
The rated voltage includes a safety margin against the electrolyte's breakdown voltage at which the electrolyte decomposes. The breakdown voltage decomposes the separating solvent molecules in the Helmholtz double-layer, e.g. water splits into hydrogen and oxygen. The solvent molecules then cannot separate the electrical charges from each other. Higher voltages than rated voltage cause hydrogen gas formation or a short circuit.
Standard supercapacitors with aqueous electrolyte normally are specified with a rated voltage of 2.1 to 2.3 V and capacitors with organic solvents with 2.5 to 2.7 V. Lithium-ion capacitors with doped electrodes may reach a rated voltage of 3.8 to 4 V, but have a low voltage limit of about 2.2 V. Supercapacitors with ionic electrolytes can exceed an operating voltage of 3.5 V.[83]
Operating supercapacitors below the rated voltage improves the long-time behavior of the electrical parameters. Capacitance values and internal resistance during cycling are more stable and lifetime and charge/discharge cycles may be extended.[87]
Higher application voltages require connecting cells in series. Since each component has a slight difference in capacitance value and ESR, it is necessary to actively or passively balance them to stabilize the applied voltage. Passive balancing employs resistors in parallel with the supercapacitors. Active balancing may include electronic voltage management above a threshold that varies the current.
Internal resistance
[edit]
Charging/discharging a supercapacitor is connected to the movement of charge carriers (ions) in the electrolyte across the separator to the electrodes and into their porous structure. Losses occur during this movement that can be measured as the internal DC resistance.
With the electrical model of cascaded, series-connected RC (resistor/capacitor) elements in the electrode pores, the internal resistance increases with the increasing penetration depth of the charge carriers into the pores. The internal DC resistance is time dependent and increases during charge/discharge. In applications often only the switch-on and switch-off range is interesting. The internal resistance Ri can be calculated from the voltage drop ΔV2 at the time of discharge, starting with a constant discharge current Idischarge. It is obtained from the intersection of the auxiliary line extended from the straight part and the time base at the time of discharge start (see picture right). Resistance can be calculated by:
The discharge current Idischarge for the measurement of internal resistance can be taken from the classification according to IEC 62391-1.
This internal DC resistance Ri should not be confused with the internal AC resistance called equivalent series resistance (ESR) normally specified for capacitors. It is measured at 1 kHz. ESR is much smaller than DC resistance. ESR is not relevant for calculating supercapacitor inrush currents or other peak currents.
Ri determines several supercapacitor properties. It limits the charge and discharge peak currents as well as charge/discharge times. Ri and the capacitance C results in the time constant
This time constant determines the charge/discharge time. A 100 F capacitor with an internal resistance of 30 mΩ for example, has a time constant of 0.03 • 100 = 3 s. After 3 seconds charging with a current limited only by internal resistance, the capacitor has 63.2% of full charge (or is discharged to 36.8% of full charge).
Standard capacitors with constant internal resistance fully charge during about 5 τ. Since internal resistance increases with charge/discharge, actual times cannot be calculated with this formula. Thus, charge/discharge time depends on specific individual construction details.
Current load and cycle stability
[edit]Because supercapacitors operate without forming chemical bonds, current loads, including charge, discharge and peak currents are not limited by reaction constraints. Current load and cycle stability can be much higher than for rechargeable batteries. Current loads are limited only by internal resistance, which may be substantially lower than for batteries.
Internal resistance "Ri" and charge/discharge currents or peak currents "I" generate internal heat losses "Ploss" according to:
This heat must be released and distributed to the ambient environment to maintain operating temperatures below the specified maximum temperature.
Heat generally defines capacitor lifetime due to electrolyte diffusion. The heat generation coming from current loads should be smaller than 5 to 10 K at maximum ambient temperature (which has only minor influence on expected lifetime). For that reason the specified charge and discharge currents for frequent cycling are determined by internal resistance.
The specified cycle parameters under maximal conditions include charge and discharge current, pulse duration and frequency. They are specified for a defined temperature range and over the full voltage range for a defined lifetime. They can differ enormously depending on the combination of electrode porosity, pore size and electrolyte. Generally a lower current load increases capacitor life and increases the number of cycles. This can be achieved either by a lower voltage range or slower charging and discharging.[87]
Supercapacitors (except those with polymer electrodes) can potentially support more than one million charge/discharge cycles without substantial capacity drops or internal resistance increases. Beneath the higher current load is this the second great advantage of supercapacitors over batteries. The stability results from the dual electrostatic and electrochemical storage principles.
The specified charge and discharge currents can be significantly exceeded by lowering the frequency or by single pulses. Heat generated by a single pulse may be spread over the time until the next pulse occurs to ensure a relatively small average heat increase. Such a "peak power current" for power applications for supercapacitors of more than 1000 F can provide a maximum peak current of about 1000 A.[88] Such high currents generate high thermal stress and high electromagnetic forces that can damage the electrode-collector connection requiring robust design and construction of the capacitors.
Device capacitance and resistance dependence on operating voltage and temperature
[edit]
Device parameters such as capacitance initial resistance and steady state resistance are not constant, but are variable and dependent on the device's operating voltage. Device capacitance will have a measurable increase as the operating voltage increases. For example: a 100F device can be seen to vary 26% from its maximum capacitance over its entire operational voltage range. Similar dependence on operating voltage is seen in steady state resistance (Rss) and initial resistance (Ri).[89] Device properties can also be seen to be dependent on device temperature. As the temperature of the device changes either through operation of varying ambient temperature, the internal properties such as capacitance and resistance will vary as well. Device capacitance is seen to increase as the operating temperature increases.[89]
Energy capacity
[edit]
Supercapacitors occupy the gap between high power/low energy electrolytic capacitors and low power/high energy rechargeable batteries. The energy Wmax (expressed in Joule) that can be stored in a capacitor is given by the formula
This formula describes the amount of energy stored and is often used to describe new research successes. However, only part of the stored energy is available to applications, because the voltage drop and the time constant over the internal resistance mean that some of the stored charge is inaccessible. The effective realized amount of energy Weff is reduced by the used voltage difference between Vmax and Vmin and can be represented as:[citation needed]
This formula also represents the energy asymmetric voltage components such as lithium ion capacitors.
Specific energy and specific power
[edit]The amount of energy that can be stored in a capacitor per mass of that capacitor is called its specific energy. Specific energy is measured gravimetrically (per unit of mass) in watt-hours per kilogram (Wh/kg).
The amount of energy can be stored in a capacitor per volume of that capacitor is called its energy density (also called volumetric specific energy in some literature). Energy density is measured volumetrically (per unit of volume) in watt-hours per litre (Wh/L). Units of liters and dm3 can be used interchangeably.
As of 2013[update] commercial energy density varies widely, but in general range from around 5 to 8 Wh/L. In comparison, petrol fuel has an energy density of 32.4 MJ/L or 9000 Wh/L.[90] Commercial specific energies range from around 0.5 to 15 Wh/kg. For comparison, an aluminum electrolytic capacitor stores typically 0.01 to 0.3 Wh/kg, while a conventional lead-acid battery stores typically 30 to 40 Wh/kg and modern lithium-ion batteries 100 to 265 Wh/kg. Supercapacitors can therefore store 10 to 100 times more energy than electrolytic capacitors, but only one tenth as much as batteries.[citation needed] For reference, petrol fuel has a specific energy of 44.4 MJ/kg or 12300 Wh/kg.
Although the specific energy of supercapacitors is defavorably compared with batteries, capacitors have the important advantage of the specific power. Specific power describes the speed at which energy can be delivered to the load (or, in charging the device, absorbed from the generator). The maximum power Pmax specifies the power of a theoretical rectangular single maximum current peak of a given voltage. In real circuits the current peak is not rectangular and the voltage is smaller, caused by the voltage drop, so IEC 62391–2 established a more realistic effective power Peff for supercapacitors for power applications, which is half the maximum and given by the following formulas :
- ,
with V = voltage applied and Ri, the internal DC resistance of the capacitor.
Just like specific energy, specific power is measured either gravimetrically in kilowatts per kilogram (kW/kg, specific power) or volumetrically in kilowatts per litre (kW/L, power density).Supercapacitor specific power is typically 10 to 100 times greater than for batteries and can reach values up to 15 kW/kg.
Ragone charts relate energy to power and are a valuable tool for characterizing and visualizing energy storage components. With such a diagram, the position of specific power and specific energy of different storage technologies is easily to compare, see diagram.[91][92]
Lifetime
[edit]
Since supercapacitors do not rely on chemical changes in the electrodes (except for those with polymer electrodes), lifetimes depend mostly on the rate of evaporation of the liquid electrolyte. This evaporation is generally a function of temperature, current load, current cycle frequency and voltage. Current load and cycle frequency generate internal heat, so that the evaporation-determining temperature is the sum of ambient and internal heat. This temperature is measurable as core temperature in the center of a capacitor body. The higher the core temperature, the faster the evaporation, and the shorter the lifetime.
Evaporation generally results in decreasing capacitance and increasing internal resistance. According to IEC/EN 62391-2, capacitance reductions of over 30%, or internal resistance exceeding four times its data sheet specifications, are considered "wear-out failures," implying that the component has reached end-of-life. The capacitors are operable, but with reduced capabilities. Whether the aberration of the parameters have any influence on the proper functionality depends on the application of the capacitors.
Such large changes of electrical parameters specified in IEC/EN 62391-2 are usually unacceptable for high current load applications. Components that support high current loads use much smaller limits, e.g., 20% loss of capacitance or double the internal resistance.[93] The narrower definition is important for such applications, since heat increases linearly with increasing internal resistance, and the maximum temperature should not be exceeded. Temperatures higher than specified can destroy the capacitor.
The real application lifetime of supercapacitors, also called "service life," "life expectancy," or "load life," can reach 10 to 15 years or more, at room temperature. Such long periods cannot be tested by manufacturers. Hence, they specify the expected capacitor lifetime at the maximum temperature and voltage conditions. The results are specified in datasheets using the notation "tested time (hours)/max. temperature (°C)," such as "5000 h/65 °C". With this value, and expressions derived from historical data, lifetimes can be estimated for lower temperature conditions.
Datasheet lifetime specification is tested by the manufactures using an accelerated aging test called an "endurance test," with maximum temperature and voltage over a specified time. For a "zero defect" product policy, no wear out or total failure may occur during this test.
The lifetime specification from datasheets can be used to estimate the expected lifetime for a given design. The "10-degrees-rule" used for electrolytic capacitors with non-solid electrolyte is used in those estimations, and can be used for supercapacitors. This rule employs the Arrhenius equation: a simple formula for the temperature dependence of reaction rates. For every 10 °C reduction in operating temperature, the estimated life doubles.
With:
- Lx = estimated lifetime
- L0 = specified lifetime
- T0 = upper specified capacitor temperature
- Tx = actual operating temperature of the capacitor cell
Calculated with this formula, capacitors specified with 5000 h at 65 °C, have an estimated lifetime of 20,000 h at 45 °C.
Lifetimes are also dependent on the operating voltage, because the development of gas in the liquid electrolyte depends on the voltage. The lower the voltage, the smaller the gas development, and the longer the lifetime. No general formula relates voltage to lifetime. The voltage dependent curves shown from the picture are an empirical result from one manufacturer.
Life expectancy for power applications may be also limited by current load or number of cycles. This limitation has to be specified by the relevant manufacturer and is strongly type dependent.
Self-discharge
[edit]Storing electrical energy in the double-layer separates the charge carriers within the pores by distances in the range of molecules. Irregularities can occur over this short distance, leading to a small exchange of charge carriers and gradual discharge. This self-discharge is called leakage current. Leakage depends on capacitance, voltage, temperature, and the chemical stability of the electrode/electrolyte combination. At room temperature, leakage is so low that it is specified as time to self-discharge in hours, days, or weeks. As an example, a 5.5 V/F Panasonic "Goldcapacitor" specifies a voltage drop at 20 °C from 5.5 V to 3 V in 600 hours (25 days or 3.6 weeks) for a double cell capacitor.[94]
Post charge voltage relaxation
[edit]
It has been noticed that after the EDLC experiences a charge or discharge, the voltage will drift over time, relaxing toward its previous voltage level. The observed relaxation can occur over several hours and is likely due to long diffusion time constants of the porous electrodes within the EDLC.[89]
Polarity
[edit]Since the positive and negative electrodes (or simply positrode and negatrode, respectively) of symmetric supercapacitors consist of the same material, theoretically supercapacitors have no true polarity and catastrophic failure does not normally occur. However reverse-charging a supercapacitor lowers its capacity, so it is recommended practice to maintain the polarity resulting from the formation of the electrodes during production. Asymmetric supercapacitors are inherently polar.
Pseudocapacitor and hybrid supercapacitors which have electrochemical charge properties may not be operated with reverse polarity, precluding their use in AC operation. However, this limitation does not apply to EDLC supercapacitors
A bar in the insulating sleeve identifies the negative terminal in a polarized component.
In some literature, the terms "anode" and "cathode" are used in place of negative electrode and positive electrode. Using anode and cathode to describe the electrodes in supercapacitors (and also rechargeable batteries, including lithium-ion batteries) can lead to confusion, because the polarity changes depending on whether a component is considered as a generator or as a consumer of current. In electrochemistry, cathode and anode are related to reduction and oxidation reactions, respectively. However, in supercapacitors based on electric double-layer capacitance, there is no oxidation nor reduction reactions on any of the two electrodes. Therefore, the concepts of cathode and anode do not apply.
Comparison of selected commercial supercapacitors
[edit]The range of electrodes and electrolytes available yields a variety of components suitable for diverse applications. The development of low-ohmic electrolyte systems, in combination with electrodes with high pseudocapacitance, enable many more technical solutions.
The following table shows differences among capacitors of various manufacturers in capacitance range, cell voltage, internal resistance (ESR, DC or AC value) and volumetric and gravimetric specific energy. In the table, ESR refers to the component with the largest capacitance value of the respective manufacturer. Roughly, they divide supercapacitors into two groups. The first group offers greater ESR values of about 20 milliohms and relatively small capacitance of 0.1 to 470 F. These are "double-layer capacitors" for memory back-up or similar applications. The second group offers 100 to 10,000 F with a significantly lower ESR value under 1 milliohm. These components are suitable for power applications. A correlation of some supercapacitor series of different manufacturers to the various construction features is provided in Pandolfo and Hollenkamp.[38]
In commercial double-layer capacitors, or, more specifically, EDLCs in which energy storage is predominantly achieved by double-layer capacitance, energy is stored by forming an electrical double layer of electrolyte ions on the surface of conductive electrodes. Since EDLCs are not limited by the electrochemical charge transfer kinetics of batteries, they can charge and discharge at a much higher rate, with lifetimes of more than 1 million cycles. The EDLC energy density is determined by operating voltage and the specific capacitance (farad/gram or farad/cm3) of the electrode/electrolyte system. The specific capacitance is related to the Specific Surface Area (SSA) accessible by the electrolyte, its interfacial double-layer capacitance, and the electrode material density.
Commercial EDLCs are based on two symmetric electrodes impregnated with electrolytes comprising tetraethylammonium tetrafluoroborate salts in organic solvents. Current EDLCs containing organic electrolytes operate at 2.7 V and reach energy densities around 5-8 Wh/kg and 7 to 10 Wh/L. The specific capacitance is related to the specific surface area (SSA) accessible by the electrolyte, its interfacial double-layer capacitance, and the electrode material density. Graphene-based platelets with mesoporous spacer material is a promising structure for increasing the SSA of the electrolyte.[95]
Standards
[edit]
Supercapacitors vary sufficiently that they are rarely interchangeable, especially those with higher specific energy. Applications range from low to high peak currents, requiring standardized test protocols.[96]
Test specifications and parameter requirements are specified in the generic specification IEC/EN 62391–1, Fixed electric double layer capacitors for use in electronic equipment.
The standard defines four application classes, according to discharge current levels:
- Memory backup
- Energy storage, mainly used for driving motors require a short time operation,
- Power, higher power demand for a long time operation,
- Instantaneous power, for applications that requires relatively high current units or peak currents ranging up to several hundreds of amperes even with a short operating time
Three further standards describe special applications:
- IEC 62391–2, Fixed electric double-layer capacitors for use in electronic equipment - Blank detail specification - Electric double-layer capacitors for power application
- IEC 62576, Electric double-layer capacitors for use in hybrid electric vehicles. Test methods for electrical characteristics
- BS/EN 61881-3, Railway applications. Rolling stock equipment. Capacitors for power electronics. Electric double-layer capacitors
Applications
[edit]Supercapacitors have advantages in applications where a large amount of power is needed for a relatively short time, where a very high number of charge/discharge cycles or a longer lifetime is required. Typical applications range from milliamp currents or milliwatts of power for up to a few minutes to several amps current or several hundred kilowatts power for much shorter periods.
Supercapacitors do not support alternating current (AC) applications.
Consumer electronics
[edit]In applications with fluctuating loads, such as laptop computers, PDAs, GPS, portable media players, hand-held devices,[97] and photovoltaic systems, supercapacitors can stabilize the power supply.
Supercapacitors deliver power for photographic flashes in digital cameras and for LED flashlights that can be charged in much shorter periods of time, e.g., 90 seconds.[98]
Some portable speakers are powered by supercapacitors.[99]
A cordless electric screwdriver with supercapacitors for energy storage has about half the run time of a comparable battery model, but can be fully charged in 90 seconds. It retains 85% of its charge after three months left idle.[100]
Power generation and distribution
[edit]Grid power buffering
[edit]Numerous non-linear loads, such as EV chargers, HEVs, air conditioning systems, and advanced power conversion systems cause current fluctuations and harmonics.[101][102] These current differences create unwanted voltage fluctuations and therefore power oscillations on the grid.[101] Power oscillations not only reduce the efficiency of the grid, but can cause voltage drops in the common coupling bus, and considerable frequency fluctuations throughout the entire system. To overcome this problem, supercapacitors can be implemented as an interface between the load and the grid to act as a buffer between the grid and the high pulse power drawn from the charging station.[103][104]
Low-power equipment power buffering
[edit]
Supercapacitors provide backup or emergency shutdown power to low-power equipment such as RAM, SRAM, micro-controllers and PC Cards. They are the sole power source for low energy applications such as automated meter reading (AMR)[105] equipment or for event notification in industrial electronics.
Supercapacitors buffer power to and from rechargeable batteries, mitigating the effects of short power interruptions and high current peaks. Batteries kick in only during extended interruptions, e.g., if the mains power or a fuel cell fails, which lengthens battery life.
Uninterruptible power supplies (UPS) may be powered by supercapacitors, which can replace much larger banks of electrolytic capacitors. This combination reduces the cost per cycle, saves on replacement and maintenance costs, enables the battery to be downsized and extends battery life.[106][107][108]
Supercapacitors provide backup power for actuators in wind turbine pitch systems, so that blade pitch can be adjusted even if the main supply fails.[109]
Voltage stabilization
[edit]Supercapacitors can stabilize voltage fluctuations for powerlines by acting as dampers. Wind and photovoltaic systems exhibit fluctuating supply evoked by gusting or clouds that supercapacitors can buffer within milliseconds. [110][111]
Micro grids
[edit]Micro grids are usually powered by clean and renewable energy. Most of this energy generation, however, is not constant throughout the day and does not usually match demand. Supercapacitors can be used for micro grid storage to instantaneously inject power when the demand is high and the production dips momentarily, and to store energy in the reverse conditions. They are useful in this scenario, because micro grids are increasingly producing power in DC, and capacitors can be utilized in both DC and AC applications. Supercapacitors work best in conjunction with chemical batteries. They provide an immediate voltage buffer to compensate for quick changing power loads due to their high charge and discharge rate through an active control system.[112] Once the voltage is buffered, it is put through an inverter to supply AC power to the grid. Supercapacitors cannot provide frequency correction in this form directly in the AC grid.[113][114]
Energy harvesting
[edit]Supercapacitors are suitable temporary energy storage devices for energy harvesting systems. In energy harvesting systems, the energy is collected from the ambient or renewable sources, e.g., mechanical movement, light or electromagnetic fields, and converted to electrical energy in an energy storage device. For example, it was demonstrated that energy collected from RF (radio frequency) fields (using an RF antenna as an appropriate rectifier circuit) can be stored to a printed supercapacitor. The harvested energy was then used to power an application-specific integrated circuit (ASIC) for over 10 hours.[115]
Batteries
[edit]The UltraBattery is a hybrid rechargeable lead-acid battery and a supercapacitor. Its cell construction contains a standard lead-acid battery positive electrode, standard sulphuric acid electrolyte and a specially prepared negative carbon-based electrode that store electrical energy with double-layer capacitance. The presence of the supercapacitor electrode alters the chemistry of the battery and affords it significant protection from sulfation in high rate partial state of charge use, which is the typical failure mode of valve regulated lead-acid cells used this way. The resulting cell performs with characteristics beyond either a lead-acid cell or a supercapacitor, with charge and discharge rates, cycle life, efficiency and performance all enhanced.
Medical
[edit]Supercapacitors are used in defibrillators where they can deliver 500 joules to shock the heart back into sinus rhythm.[116]
Military
[edit]Supercapacitors' low internal resistance supports applications that require short-term high currents. Among the earliest uses were motor startup (cold engine starts, particularly with diesels) for large engines in tanks and submarines. Supercapacitors buffer the battery, handling short current peaks, reducing cycling and extending battery life. Further military applications that require high specific power are phased array radar antennae, laser power supplies, military radio communications, avionics displays and instrumentation, backup power for airbag deployment and GPS-guided missiles and projectiles.
Transport
[edit]A primary challenge of all transport is reducing energy consumption and reducing CO
2 emissions. Recovery of braking energy (recuperation or regenerative braking) helps with both. This requires components that can quickly store and release energy over long times with a high cycle rate. Supercapacitors fulfill these requirements and are therefore used in various applications in transportation.
Aviation
[edit]In 2005, aerospace systems and controls company Diehl Luftfahrt Elektronik GmbH chose supercapacitors to power emergency actuators for doors and evacuation slides used in airliners, including the Airbus 380.[109]
Cars
[edit]The Toyota Yaris Hybrid-R concept car uses a supercapacitor to provide bursts of power. PSA Peugeot Citroën has started using supercapacitors as part of its stop-start fuel-saving system, which permits faster initial acceleration.[117] Mazda's i-ELOOP system stores energy in a supercapacitor during deceleration and uses it to power on-board electrical systems while the engine is stopped by the stop-start system.
Rail
[edit]Supercapacitors can be used to supplement batteries in starter systems in diesel railroad locomotives with diesel-electric transmission. The capacitors capture the braking energy of a full stop and deliver the peak current for starting the diesel engine and acceleration of the train and ensures the stabilization of line voltage. Depending on the driving mode up to 30% energy saving is possible by recovery of braking energy. Low maintenance and environmentally friendly materials encouraged the choice of supercapacitors.[118]
Plant machinery
[edit]Mobile hybrid Diesel-electric rubber tyred gantry cranes move and stack containers within a terminal. Lifting the boxes requires large amounts of energy. Some of the energy could be recaptured while lowering the load, resulting in improved efficiency.[119] A triple hybrid forklift truck uses fuel cells and batteries as primary energy storage and supercapacitors to buffer power peaks by storing braking energy. They provide the fork lift with peak power over 30 kW. The triple-hybrid system offers over 50% energy savings compared with Diesel or fuel-cell systems.[120] Supercapacitor-powered terminal tractors transport containers to warehouses. They provide an economical, quiet and pollution-free alternative to Diesel terminal tractors.[121]
Light rail
[edit]Supercapacitors make it possible not only to reduce energy, but to replace overhead lines in historical city areas, so preserving the city's architectural heritage. This approach may allow many new light rail city lines to replace overhead wires that are too expensive to fully route.
In 2003 Mannheim adopted a prototype light-rail vehicle (LRV) using the MITRAC Energy Saver system from Bombardier Transportation to store mechanical braking energy with a roof-mounted supercapacitor unit.[122][123] It contains several units each made of 192 capacitors with 2700 F / 2.7 V interconnected in three parallel lines. This circuit results in a 518 V system with an energy content of 1.5 kWh. For acceleration when starting this "on-board-system" can provide the LRV with 600 kW and can drive the vehicle up to 1 km without overhead line supply, thus better integrating the LRV into the urban environment. Compared to conventional LRVs or Metro vehicles that return energy into the grid, onboard energy storage saves up to 30% and reduces peak grid demand by up to 50%.[124]

В 2009 году суперконденсаторы позволили LRV работать в исторической части Гейдельберга без воздушных проводов, сохраняя тем самым архитектурное наследие города. [ нужна ссылка ] Оборудование SC стоило дополнительно 270 000 евро за транспортное средство, которые, как ожидалось, окупятся в течение первых 15 лет эксплуатации. Суперконденсаторы заряжаются на остановках, когда транспортное средство находится на плановой остановке. В апреле 2011 года немецкий региональный транспортный оператор Рейн-Неккар, отвечающий за Гейдельберг, заказал еще 11 единиц. [125]
В 2009 году компании Alstom и RATP оснастили трамвай Citadis экспериментальной системой рекуперации энергии под названием «STEEM». [126] Система оснащена 48 установленными на крыше суперконденсаторами для хранения энергии торможения, что обеспечивает трамваям высокий уровень энергетической автономности, позволяя им работать без воздушных линий электропередач на некоторых участках маршрута, подзаряжаясь во время движения на промежуточных станциях с электроприводом. Во время испытаний, которые проходили между остановками Porte d'Italie и Porte de Choisy на линии T3 трамвайной сети Парижа , трамвай потреблял в среднем примерно на 16% меньше энергии. [127]

В 2012 году оператор трамвая «Женева общественный транспорт» начал испытания LRV, оснащенного прототипом установленного на крыше суперконденсатора для рекуперации энергии торможения. [128]
Siemens поставляет системы легкорельсового транспорта с суперконденсаторами, которые включают в себя мобильные хранилища. [129]
Линия метро Южного острова Гонконга будет оснащена двумя накопителями энергии мощностью 2 МВт, которые, как ожидается, снизят потребление энергии на 10%. [130]
В августе 2012 года китайская корпорация CSR Zhuzhou Electric Locomotive представила прототип двухвагонного легкого поезда метро, оснащенного установленным на крыше суперконденсаторным блоком. Поезд может проехать 2 км без проводов, заряжаясь на станциях за 30 секунд с помощью наземного пикапа. Поставщик заявил, что поезда можно будет использовать в 100 малых и средних городах Китая. [131] планировалось ввести в эксплуатацию семь трамваев (уличных вагонов), работающих на суперконденсаторах В 2014 году в Гуанчжоу , Китай, . Суперконденсаторы заряжаются за 30 секунд с помощью устройства, расположенного между рельсами. Это позволяет трамваю преодолевать расстояние до 4 километров (2,5 мили). [132] По состоянию на 2017 год суперконденсаторные транспортные средства Чжучжоу также используются в новой трамвайной системе Нанкина и проходят испытания в Ухане . [133]
В 2012 году в Лионе (Франция) SYTRAL (Управление общественного транспорта Лиона) начало эксперименты с системой «придорожной регенерации», построенной Adetel Group, которая разработала собственную энергосберегающую систему под названием «NeoGreen» для LRV, LRT и метрополитенов. [134]
В 2014 году в Китае начали использовать трамваи с суперконденсаторами, которые заряжаются за 30 секунд с помощью устройства, расположенного между рельсами, сохраняя энергию для пробега трамвая на расстояние до 4 км — более чем достаточно, чтобы добраться до следующей остановки, где цикл можно повторить. .
В 2015 году Alstom анонсировала SRS — систему хранения энергии, которая заряжает суперконденсаторы на борту трамвая с помощью проводящих рельсов, расположенных на уровне земли, расположенных на трамвайных остановках. Это позволяет трамваям работать без воздушных линий связи на короткие расстояния. [135] Система рекламировалась как альтернатива системе наземного электропитания (APS) компании или может использоваться вместе с ней, как в случае с сетью VLT в Рио-де-Жанейро , Бразилия, которая открылась в 2016 году. [136]
CAF также предлагает суперконденсаторы для своих трамваев Urbos 3 в виде системы ACR . [117]
Автобусы
[ редактировать ]
Maxwell Technologies , американский производитель суперконденсаторов, заявил, что более 20 000 гибридных автобусов используют эти устройства для увеличения ускорения, особенно в Китае. [ нужна ссылка ]
Первый гибридный электробус с суперконденсаторами в Европе появился в 2001 году в Нюрнберге , Германия. Это был так называемый «Ultracapbus» MAN, который прошел испытания в реальной эксплуатации в 2001/2002 году. Тестовый автомобиль был оснащен дизель-электрическим приводом в сочетании с суперконденсаторами. В комплект поставки системы входило 8 модулей Ultracap на 80 В, каждый из которых содержал 36 компонентов. Система работала при напряжении 640 В и могла заряжаться/разряжаться током 400 А. Ее энергоемкость составляла 0,4 кВтч при весе 400 кг.
Суперконденсаторы улавливали энергию торможения и передавали пусковую энергию. Расход топлива был снижен на 10–15% по сравнению с обычными дизельными автомобилями. Другие преимущества включали снижение выбросов CO.
2 , тихий запуск двигателя без выбросов, снижение вибрации и снижение затрат на техническое обслуживание. [137] [138]

По состоянию на 2002 год [update] в Люцерне , Швейцария, был протестирован парк электрических автобусов TOHYCO-Rider. Суперконденсаторы можно было заряжать с помощью индуктивного бесконтактного высокоскоростного зарядного устройства после каждого цикла транспортировки в течение 3–4 минут. [139]
В начале 2005 года в Шанхае были проведены испытания новой формы электрического автобуса под названием «капабус» , который работает без линий электропередачи (работа без цепной связи) с использованием больших бортовых суперконденсаторов, которые частично заряжаются всякий раз, когда автобус останавливается (под так называемыми электрическими зонтиками), и полностью заряжаются во время остановки. конечная остановка . В 2006 году кабабузы начали использовать два коммерческих автобусных маршрута; один из них — маршрут 11 в Шанхае. Было подсчитано, что автобус с суперконденсатором дешевле, чем автобус с литий-ионным аккумулятором, а затраты энергии на один из его автобусов в десять раз ниже, чем у дизельного автобуса, а экономия топлива за весь срок службы составила 200 000 долларов. [140]
Гибридный электрический автобус под названием tribrid был представлен в 2008 году Университетом Гламоргана , Уэльс , для использования в качестве студенческого транспорта. Он питается от водородного топлива или солнечных элементов , батарей и ультраконденсаторов. [141] [142]
Автогонки
[ редактировать ]
FIA правил по , руководящий орган по автоспорту, предложила в Основе регулирования силовых агрегатов для Формулы-1 версии 1.3 от 23 мая 2007 года издать новый свод силовым агрегатам , который включает гибридный привод мощностью до 200 кВт и выходная мощность с использованием «супербатарей», состоящих из батарей и суперконденсаторов, соединенных параллельно ( KERS ). [143] [144] С помощью системы KERS можно достичь около 20% эффективности от бака до колеса. Автомобиль Toyota TS030 Hybrid LMP1, гоночный автомобиль, разработанный по правилам прототипа Ле-Мана , использует гибридную трансмиссию с суперконденсаторами. [145] [146] В гонке «24 часа Ле-Мана» 2012 года TS030 квалифицировался с самым быстрым кругом всего на 1,055 секунды медленнее (3:24,842 против 3:23,787). [147] чем самый быстрый автомобиль, Audi R18 e-tron quattro с накопителем энергии на маховике . Компоненты суперконденсатора и маховика, способность которых быстро заряжаться и разряжаться помогает как в торможении, так и в ускорении, сделали гибриды Audi и Toyota самыми быстрыми автомобилями в гонке. В гонке Ле-Ман 2012 года два конкурирующих TS030, один из которых лидировал на протяжении части гонки, оба сошли с дистанции по причинам, не связанным с суперконденсаторами. TS030 выиграл три из 8 гонок сезона чемпионата мира по гонкам на выносливость FIA 2012 года . В 2014 году в Toyota TS040 Hybrid использовался суперконденсатор, чтобы добавить 480 лошадиных сил от двух электродвигателей. [132]
Гибридные электромобили
[ редактировать ]
Комбинации суперконденсатора и аккумулятора в электромобилях (EV) и гибридных электромобилях (HEV) хорошо изучены. [96] [148] [149] Заявлено, что за счет рекуперации энергии торможения в электромобилях или гибридных автомобилях можно сократить расход топлива на 20–60%. Способность суперконденсаторов заряжаться гораздо быстрее, чем батареи, их стабильные электрические свойства, более широкий температурный диапазон и более длительный срок службы подходят, но вес, объем и особенно стоимость нивелируют эти преимущества.
Низкая удельная энергия суперконденсаторов делает их непригодными для использования в качестве автономного источника энергии при движении на большие расстояния. [150] Улучшение экономии топлива при использовании конденсатора и аккумуляторной батареи составляет около 20% и доступно только для коротких поездок. При езде на дальние расстояния преимущество снижается до 6%. Транспортные средства, сочетающие в себе конденсаторы и аккумуляторы, работают только на экспериментальных автомобилях. [151]
По состоянию на 2013 год [update] Все автомобильные производители электромобилей и гибридных автомобилей разработали прототипы, в которых вместо аккумуляторов используются суперконденсаторы для хранения энергии торможения с целью повышения эффективности трансмиссии. Mazda 6 — единственный серийный автомобиль, в котором для рекуперации энергии торможения используются суперконденсаторы. Утверждается, что рекуперативное торможение, получившее название i-eloop, снижает расход топлива примерно на 10%. [152] Серия российских Ё-каров Ё-мобиль представляла собой концепт-кроссовер-гибридный автомобиль, работающий с бензиновым приводом поворотно-лопастного типа и электрогенератором для привода тяговых двигателей. Суперконденсатор с относительно низкой емкостью восстанавливает энергию торможения для питания электродвигателя при ускорении с места. [153] Концепт-кар Toyota Yaris Hybrid-R использует суперконденсатор для обеспечения быстрого увеличения мощности. [132] PSA Peugeot Citroën оснащает некоторые свои автомобили суперконденсаторами в рамках своей системы экономии топлива «стоп-старт», поскольку это позволяет быстрее запускать двигатели, когда светофор загорается зеленым. [132]
Гондолы
[ редактировать ]
В Целль-ам-Зее , Австрия , канатная дорога соединяет город с Шмиттенхёэ горой . Иногда гондолы ходят 24 часа в сутки, используя электричество для освещения, открытия дверей и связи. Единственное доступное время для подзарядки аккумуляторов на станциях — это короткие интервалы погрузки и разгрузки гостей, которые слишком коротки для подзарядки аккумуляторов. Суперконденсаторы обеспечивают быструю зарядку, большее количество циклов и более длительный срок службы, чем батареи. Emirates Air Line (канатная дорога) , также известная как канатная дорога Темзы, — это гондольная линия длиной 1 километр (0,62 мили) в Лондоне , Великобритания , которая пересекает Темзу от полуострова Гринвич до Королевских доков . Кабины оснащены современной информационно-развлекательной системой, работающей на суперконденсаторах. [154] [155]
События
[ редактировать ]По состоянию на 2013 год [update] коммерчески доступные литий-ионные суперконденсаторы предлагали самую высокую на сегодняшний день весовую удельную энергию, достигающую 15 Втч/кг ( 54 кДж/кг ). Исследования направлены на улучшение удельной энергии, снижение внутреннего сопротивления, расширение температурного диапазона, увеличение срока службы и снижение затрат. [22] Проекты включают электроды с индивидуальным размером пор, псевдоемкостные покрытия или легирующие материалы, а также улучшенные электролиты.
Разработка | Дата | Удельная энергия [А] | Удельная мощность | Циклы | Емкость | Примечания |
---|---|---|---|---|---|---|
Листы графена, сжатые капиллярным сжатием летучей жидкости [156] | 2013 | 60 Втч /л | Интеграция электролитов субнанометрового масштаба создала непрерывную сеть транспорта ионов. | |||
Вертикально ориентированные электроды из углеродных нанотрубок [10] [60] | 2007 2009 2013 | 13,50 Втч /кг | 37,12 Вт /г | 300,000 | Первая реализация [157] | |
Изогнутые листы графена [55] [56] | 2010 | 85,6 Втч /кг | 550 Ф /г | Однослойные изогнутые листы графена, которые не укладываются друг на друга, образуя мезопоры, доступные и смачиваемые экологически чистыми ионными электролитами при напряжении до 4 В. | ||
KOH реструктурированный оксид графита [158] [159] | 2011 | 85 Втч /кг | >10 000 | 200 Ф /г | Гидроксид калия реструктурировал углерод, образовав трехмерную пористую сетку. | |
Активированные угли на основе графена как электроды суперконденсаторов с макро- и мезопорами [160] | 2013 | 74 Втч /кг | Трехмерные пористые структуры в углеродах, полученных из графена, в которых мезопоры интегрированы в макропористые каркасы с площадью поверхности 3290 м². 2 /г | |||
Сопряженный микропористый полимер [161] [162] | 2011 | 53 Втч /кг | 10,000 | Аза-слитый π-сопряженный микропористый каркас | ||
SWNT композитный электрод [163] | 2011 | 990 Вт /кг | Специально разработанная мезо-макропористая структура удерживает больше электролита, обеспечивая легкий транспорт ионов. | |||
Наночешуйки гидроксида никеля на композитном электроде из УНТ [164] | 2012 | 50,6 Втч /кг | 3300 Ф /г | Асимметричный суперконденсатор, использующий электрод Ni(OH) 2 /CNT/NF в качестве анода в сочетании с катодом из активированного угля (AC), обеспечивающий напряжение ячейки 1,8 В. | ||
Наногибрид аккумулятор-электрод [76] | 2012 | 40 Втч /л | 7,5 Вт /л | 10,000 | Что 4 Ти 5 О 12 (LTO), нанесенный на анод из углеродных нановолокон (CNF) и катод из активированного угля. | |
Никель- кобальтит, нанесенный на мезопористый углеродный аэрогель [165] | 2012 | 53 Втч /кг | 2,25 Вт /кг | 1700 Ф /г | Никель-кобальтит — недорогой и экологически чистый суперемкостный материал. | |
диоксида марганца Интеркалированные нанохлопья [166] | 2013 | 110 Втч /кг | 1000 Ф /г | Мокрый электрохимический процесс интеркалировал ионы Na(+) в MnO. 2 прослойки. Наночешуйчатые электроды демонстрируют более быструю ионную диффузию с усиленными окислительно-восстановительными пиками. | ||
3D-пористый графеновый электрод [167] | 2013 | 98 Втч /кг | 231 Ф /г | Морщинистые однослойные листы графена размером в несколько нанометров, по крайней мере, с некоторыми ковалентными связями. | ||
Планарные микросуперконденсаторы на основе графена для хранения энергии на кристалле [168] | 2013 | 2,42 Втч /л | Фильтрация на чип-линии | |||
Нанолистовые конденсаторы [169] [170] | 2014 | 27,5 мкФ см −2 | Электроды: Ру 0,95 О 2 0.2– Диэлектрик: Ca 2 Nb 3 O 10 –. Производственные процессы на основе растворов при комнатной температуре. Общая толщина менее 30 нм. | |||
LSG/диоксид марганца [171] | 2015 | 42 Втч/л | 10 кВт/л | 10,000 | Трехмерная структура графена (LSG), нанесенная лазером, для определения проводимости, пористости и площади поверхности. Электроды имеют толщину около 15 микрон. | |
Лазерно-индуцированный графен/твердотельный электролит [172] [173] | 2015 | 0,02 мА/см 2 | 9 мФ/см 2 | Выдерживает многократное сгибание. | ||
Нанопроволоки триоксида вольфрама (WO 3 ) и двумерные оболочки из дихалькогенида переходного металла , дисульфида вольфрама (WS 2 ) [174] [175] | 2016 | ~100 Втч/л | 1 кВт/л | 30,000 | 2D-оболочки, окружающие нанопроволоки |
А Исследование электродных материалов требует измерения отдельных компонентов, таких как электрод или полуэлемент. [176] Используя противоэлектрод, который не влияет на измерения, можно выявить характеристики только интересующего электрода. Удельная энергия и мощность реальных суперконденсаторов составляют примерно 1/3 от плотности электродов.
Рынок
[ редактировать ]По состоянию на 2016 год [update] Мировые продажи суперконденсаторов составляют около 400 миллионов долларов США. [177]
Рынок аккумуляторов (по оценкам Frost & Sullivan ) вырос с 47,5 миллиардов долларов США (76,4% или 36,3 миллиардов долларов США из которых приходилось на аккумуляторные батареи) до 95 миллиардов долларов США. [178] Рынок суперконденсаторов по-прежнему представляет собой небольшую нишу, которая не поспевает за своим более крупным конкурентом.
В 2016 году IDTechEx прогнозирует, что к 2026 году продажи вырастут с 240 миллионов долларов до 2 миллиардов долларов, то есть годовой прирост составит около 24%. [179]
Стоимость суперконденсаторов в 2006 году составляла 0,01 доллара США за фарад или 2,85 доллара США за килоджоуль, а в 2008 году опустилась ниже 0,01 доллара США за фарад и, как ожидается, в среднесрочной перспективе продолжит снижаться. [180]
См. также
[ редактировать ]- Транспортное средство Capa — тип транспортного средства.
- Типы конденсаторов . Стили производства электронного устройства.
- Сопряженный микропористый полимер - тип пористого материала.
- Аккумулятор электромобиля - аккумулятор, используемый для питания электродвигателей аккумуляторного электромобиля или гибридного электромобиля.
- Хранение энергии на маховике - метод хранения энергии.
- Список новых технологий : новые технологии активно разрабатываются.
- Литий-ионный конденсатор - конденсатор гибридного типа.
- Фонарик с механическим приводом - Фонарик с приводом от человека
- Наноцветок - соединение, в результате которого образуются образования, которые под микроскопом напоминают цветы.
Ссылки
[ редактировать ]- ^ Ци, Чжаосян; Кениг, Гэри М. (июль 2017 г.). «Обзорная статья: Проточные аккумуляторные системы с твердыми электроактивными материалами» . Журнал вакуумной науки и технологий B, Нанотехнологии и микроэлектроника: материалы, обработка, измерения и явления . 35 (4): 040801. Бибкод : 2017JVSTB..35d0801Q . дои : 10.1116/1.4983210 . ISSN 2166-2746 .
- ^ Хэггстрем, Фредрик; Дельсинг, Джеркер (27 ноября 2018 г.). «Хранение энергии IoT — прогноз» . Сбор энергии и системы . 5 (3–4): 43–51. дои : 10.1515/ehs-2018-0010 . S2CID 64526195 . Проверено 30 октября 2020 г. .
- ^ Буэно, Пауло Р. (28 февраля 2019 г.). «Наномасштабное происхождение явлений суперемкости». Журнал источников энергии . 414 : 420–434. Бибкод : 2019JPS...414..420B . дои : 10.1016/j.jpowsour.2019.01.010 . ISSN 0378-7753 . S2CID 104416995 .
- ^ Тегерани, З.; Томас, диджей; Корочкина Т.; Филлипс, Колорадо; Лупо, Д.; Лехтимяки, С.; О'Махони, Дж.; Гетин, DT (2 января 2017 г.). «Технология печатных суперконденсаторов большой площади для недорогого внутреннего хранения экологически чистой энергии» (PDF) . Энергия . 118 : 1313–1321. Бибкод : 2017Ene...118.1313T . дои : 10.1016/j.energy.2016.11.019 . ISSN 0360-5442 . S2CID 55090490 .
- ^ Справочник Линдена по батареям, четвертое издание . Макгроу-Хилл Образование. 21 января 2024 г. ISBN 978-0-07-162421-3 .
- ^ US 2800616 , Becker, HI, «Электролитический конденсатор низкого напряжения», выпущен 23 июля 1957 г.
- ^ Хо, Дж.; Джоу, Р.; Боггс, С. (январь 2010 г.). «Историческое введение в технологию конденсаторов» (PDF) . Журнал IEEE по электроизоляции . 26 (1): 20–25. дои : 10.1109/mei.2010.5383924 . S2CID 23077215 .
- ^ Краткая история суперконденсаторов ОСЕНЬ 2007 г. Батареи и технологии хранения энергии. Архивировано 6 января 2014 г. в Wayback Machine.
- ^ US 3288641 , Райтмайр, Роберт А., «Аппарат для хранения электрической энергии», выдан 29 ноября 1966 г.
- ^ Перейти обратно: а б с д и Дж. Г. Шиндалл, Изменение ультраконденсаторов, IEEE Spectrum, ноябрь 2007 г. [1]
- ^ США 3536963 , DL Boos, «Электролитический конденсатор с электродами из угольной пасты», выдан 27 октября 1970 г.
- ^ Перейти обратно: а б с д и ж Конвей, Брайан Эванс (1999), Электрохимические суперконденсаторы: научные основы и технологические применения (на немецком языке), Берлин, Германия: Springer , стр. 1–8, ISBN. 978-0306457364
- ^ Перейти обратно: а б Конвей, Брайан Эванс (май 1991 г.). «Переход от поведения «суперконденсатора» к поведению «батареи» при электрохимическом хранении энергии» . Дж. Электрохим. Соц . 138 (6): 1539–1548. Бибкод : 1991JElS..138.1539C . дои : 10.1149/1.2085829 .
- ^ Panasonic, Электрический двухслойный конденсатор, Техническое руководство, 1. Введение, Panasonic Goldcaps. Архивировано 9 января 2014 г. в Wayback Machine.
- ^ «Электрические двухслойные конденсаторы» . ЭЛНА. Архивировано из оригинала 13 марта 2015 года . Проверено 21 февраля 2015 г.
- ^ Перейти обратно: а б с д и ж г Адам Маркус Намисник. Обзор технологии электрохимических суперконденсаторов (PDF) (Технический отчет). Архивировано из оригинала (PDF) 22 декабря 2014 года . Проверено 21 февраля 2015 г.
- ^ США 5369547 , Дэвид А. Эванс, «Контейнеры с анодами и катодами с электролитами», выдан 29 ноября 1994 г.
- ^ Дэвид А. Эванс (Evans Company): Электролитически-электрохимический гибридный конденсатор с высокой плотностью энергии. Архивировано 4 марта 2016 года в Wayback Machine в: Материалы 14-го симпозиума по технологиям конденсаторов и резисторов. 22 марта 1994 г.
- ^ Evans Capacitor Company, серия Capattery, 2007 г. Архивировано 15 июня 2017 г. в Wayback Machine.
- ^ Дэвид А. Эванс: Самый маленький большой конденсатор - гибрид Эванса. Архивировано 3 марта 2016 г. в техническом документе Wayback Machine , Evans Capacitor Company, 2007 г.
- ^ «ФДК, Корпоративная информация, История ФДК 2000-е» . ФДК . Проверено 21 февраля 2015 г.
- ^ Перейти обратно: а б Наой, К.; Саймон, П. (весна 2008 г.). «Новые материалы и новые конфигурации для усовершенствованных электрохимических конденсаторов» (PDF) . Интерфейс . 17 (1): 34–37.
- ^ Перейти обратно: а б с д и Fracowiak, Эльжбета ; Беген, Франсуа (май 2001 г.). «Углеродные материалы для электрохимического хранения энергии в конденсаторах». Карбон . 39 (6): 937–950. Бибкод : 2001Carbo..39..937F . дои : 10.1016/S0008-6223(00)00183-4 .
- ^ Перейти обратно: а б с д и Халпер, Марин С.; Элленбоген, Джеймс К. (март 2006 г.). «Суперконденсаторы: краткий обзор» (PDF) . Группа компаний МИТЕР Наносистемы . Проверено 16 февраля 2015 г.
- ^ «Двойной электрический слой» . 2011. Архивировано из оригинала 31 мая 2011 года . Проверено 20 января 2014 г.
- ^ Шринивасан, С. (2006). «2. Интерфейсы электрод/электролит: структура и кинетика переноса заряда» . Топливные элементы: от основ к применению . Электронные книги Спрингера. ISBN 978-0-387-35402-6 .
- ^ Бутко, Алексей В.; Бутко Владимир Юрьевич; Кумзеров, Юрий А. (2023). «Общий верхний предел емкости и его проявление для интерфейсов с водным графеном» . Международный журнал молекулярных наук . 24 (13): 10861. doi : 10.3390/ijms241310861 . ПМЦ 10341730 . ПМИД 37446037 .
- ^ Ю, ГЛ; Джалиль Р.; Белль, Б.; Майоров А.С.; Блейк, П.; Щедин, Ф.; Морозов С.В.; Пономаренко, Л.А.; Кьяппини, Ф.; Видманн, С.; Цейтлер, У.; Кацнельсон, Мичиган; Гейм, АК; Новоселов К.С.; Элиас, округ Колумбия (февраль 2013 г.). «Явления взаимодействия в графене, наблюдаемые через квантовую емкость» . ПНАС . 110 (9): 3282–3286. arXiv : 1302.3967 . Бибкод : 2013PNAS..110.3282Y . дои : 10.1073/pnas.1300599110 . ПМК 3587260 . ПМИД 23401538 .
- ^ Перейти обратно: а б с д Конвей, Брайан Эванс , «Электрохимические конденсаторы — их природа, функции и применение» , Энциклопедия электрохимии , заархивировано из оригинала 13 августа 2012 г.
- ^ Fracowiak, Эльжбета ; Юревич, К.; Дельпе, К.; Беген, Франсуа (июль 2001 г.). «Нанотрубчатые материалы для суперконденсаторов». J. Источники питания . 97–98: 822–825. Бибкод : 2001JPS....97..822F . дои : 10.1016/S0378-7753(01)00736-4 .
- ^ Гартуэйт, Джози (12 июля 2011 г.). «Как работают ультраконденсаторы (и почему они терпят неудачу)» . Земля2Тех . Сеть ГигаОМ. Архивировано из оригинала 22 ноября 2012 года . Проверено 23 февраля 2015 г.
- ^ Перейти обратно: а б с Чоданкар, Нилеш Р.; Фам, Хонг Дык; Нанджундан, Ашок Кумар; Фернандо, Джозеф Ф.С.; Джаярамулу, Коллебойина; Гольберг, Дмитрий; Хан, Ён-Кю; Дубал, Дипак П. (сентябрь 2020 г.). «Истинное значение псевдоконденсаторов и показателей их производительности: асимметричные и гибридные суперконденсаторы» (PDF) . Маленький . 16 (37): e2002806. дои : 10.1002/smll.202002806 . ПМИД 32761793 . S2CID 225371915 .
- ^ Рани, младший; Тангавел, Р.; О, СИ; Ли, Ю.С.; Джанг, Дж. Х. (2019). «Суперконденсатор сверхвысокой плотности энергии; изготовление на основе свитков оксида графена, функционализированного тиолом» . Наноматериалы . 9 (2): 148. дои : 10.3390/nano9020148 . ПМК 6409971 . ПМИД 30682829 .
- ^ Ю, Л.П.; Чен, GZ (2016). «Окислительно-восстановительные электродные материалы для суперкапаттерий» (PDF) . J. Источники питания . 326 : 604–612. Бибкод : 2016JPS...326..604Y . дои : 10.1016/j.jpowsour.2016.04.095 . Архивировано из оригинала (PDF) 19 июля 2018 года . Проверено 4 декабря 2018 г.
- ^ Мальмберг, Сирет (23 сентября 2020 г.). «Электрохимическая оценка электродов на углеродной основе, полученных методом электропрядения методом прямого электропрядения, в различных неводных электролитах для хранения энергии» . Журнал углеродных исследований . 6 .
- ^ Мальмберг, Сирет; Арулепп, Мати; Савест, Наталья; Тарасова Эльвира; Васильева, Виктория; Краснов, Илья; Кяярик, Майке; Микли, Вальдек; Крумме, Андрес (1 января 2020 г.). «Непосредственно электроформованные электроды для электрических двухслойных конденсаторов из карбидного углерода» . Журнал электростатики . 103 : 103396. doi : 10.1016/j.elstat.2019.103396 . ISSN 0304-3886 .
- ^ «Могут ли нанолисты из конопли заменить графен и создать лучшие электроды для суперконденсаторов?» . Курцвейл «Ускорение интеллекта». 14 августа 2014 года . Проверено 28 февраля 2015 г.
- ^ Перейти обратно: а б с д Пандольфо, АГ; Холленкамп, AF (июнь 2006 г.). «Свойства углерода и их роль в суперконденсаторах». J. Источники питания . 157 (1): 11–27. Бибкод : 2006JPS...157...11P . дои : 10.1016/j.jpowsour.2006.02.065 .
- ^ Ким Киносита (июнь 1992 г.). Электрохимическая кислородная технология . Уайли. ISBN 978-0-471-57043-1 .
- ^ Перейти обратно: а б с «ЭнтеросорбУ, FAQ» . Карбон-Украина. 2015.
- ^ US 6787235 , Nesbitt, CC & Sun, X., «Консолидированные аморфные углеродные материалы, их производство и использование», выдан 7 сентября 2004 г., передан Reticle, Inc.
- ^ Лейн, Дж.; Юнес, С. (1992). «Влияние метода приготовления на распределение пор активированного угля из скорлупы кокосового ореха по размерам». Карбон . 30 (4): 601–604. Бибкод : 1992Carbo..30..601L . дои : 10.1016/0008-6223(92)90178-Y .
- ^ Фишер, Ю.; Салигер, Р.; Бок, В.; Петричевич, Р.; Фрике, Дж. (октябрь 1997 г.). «Углеродные аэрогели как электродный материал в суперконденсаторах». Дж. Пористый мат . 4 (4): 281–285. дои : 10.1023/А:1009629423578 . S2CID 91596134 .
- ^ Лернер, Э.Дж. (октябрь 2004 г.). «Меньше значит больше с аэрогелями: лабораторное любопытство приводит к практическому использованию» (PDF) . Промышленный физик . Американский институт физики. стр. 26–30. Архивировано из оригинала (PDF) 2 апреля 2015 года . Проверено 28 февраля 2015 г.
- ^ Лаклер, М. (1 февраля 2003 г.). «Замена накопителей энергии суперконденсаторами из углеродного аэрогеля» . Силовая электроника . Пентон. Архивировано из оригинала 21 октября 2021 года . Проверено 28 февраля 2015 г.
- ^ Чиен, Син-Чи; Ченг, Вэй-Юнь; Ван, Юн-Хуэй; Лу, Ши-Юань (5 декабря 2012 г.). «Сверхвысокие удельные емкости суперконденсаторов, достигаемые с помощью композитов никель-кобальтит/углеродный аэрогель». Передовые функциональные материалы . 22 (23): 5038–5043. дои : 10.1002/adfm.201201176 . ISSN 1616-3028 . S2CID 97810530 .
- ^ Прессер, В.; Хон, М.; Гогоци, Ю. (март 2011 г.). «Углерод, полученный из карбидов - от пористых сетей к нанотрубкам и графену». Адв. Функц. Мэтр . 21 (5): 810–833. дои : 10.1002/adfm.201002094 . S2CID 96797238 .
- ^ Коренблит Ю.; Роуз, М.; Кокрик, Э.; Борхардт, Л.; Квит, А.; Каскель, С.; Юшин Г. (февраль 2010 г.). «Высокоемкостные электрохимические конденсаторы на основе упорядоченного мезопористого углерода на основе карбида кремния» (PDF) . АСУ Нано . 4 (3): 1337–1344. дои : 10.1021/nn901825y . ПМИД 20180559 . Архивировано из оригинала (PDF) 10 января 2014 года . Проверено 16 мая 2013 г.
- ^ «Высокоэнергетические ультраконденсаторы SkelCap — технический паспорт» (PDF) . Скелетные технологии. Архивировано из оригинала (PDF) 2 апреля 2016 года . Проверено 28 февраля 2015 г.
- ^ Йоу, Джей-Джей; Балакришнан, К.; Хуанг, Дж.; Менье, В.; Самптер, Б.Г.; Шривастава, А.; Конвей, М.; Редди, АЛМ; Ю, Дж.; Вайтай, Р.; Аджаян, премьер-министр (март 2011 г.). «Ультратонкие планарные графеновые суперконденсаторы» . Нано-буквы . 11 (4): 1423–1427. Бибкод : 2011NanoL..11.1423Y . дои : 10.1021/nl200225j . ПМИД 21381713 .
- ^ Паланисельвам, Тангавелу; Пэк, Чон Бом (2015). «2D-материалы на основе графена для суперконденсаторов». 2D материалы . 2 (3): 032002. Бибкод : 2015TDM.....2c2002P . дои : 10.1088/2053-1583/2/3/032002 . S2CID 135679359 .
- ^ Пушпарадж, В.Л.; Шайджумон, ММ; Кумар, А.; Муругесан, С.; Си, Л.; Вайтай, Р.; Линхардт, Р.Дж.; Наламасу, О.; Аджаян, премьер-министр (август 2007 г.). «Гибкие накопители энергии на основе нанокомпозитной бумаги» . Учеб. Натл. акад. наук. США . 104 (34): 13574–13577. Бибкод : 2007PNAS..10413574P . дои : 10.1073/pnas.0706508104 . ЧВК 1959422 . ПМИД 17699622 .
- ^ Маркус, Дж. (15 марта 2012 г.). «Исследователи разрабатывают графеновый суперконденсатор, перспективный для портативной электроники» . ФизОрг . Сеть Science X. Проверено 28 февраля 2015 г.
- ^ Эль-Кади, МФ; Стронг, В.; Дубин, С.; Канер, РБ (март 2012 г.). «Лазерное скрайбирование высокопроизводительных и гибких электрохимических конденсаторов на основе графена» . Наука . 335 (6074): 1326–1330. Бибкод : 2012Sci...335.1326E . дои : 10.1126/science.1216744 . ПМИД 22422977 . S2CID 18958488 .
- ^ Перейти обратно: а б Дюме, Б. (26 ноября 2010 г.). «Графеновый суперконденсатор побил рекорд емкости» . Мир физики . ИОП . Проверено 28 февраля 2015 г.
- ^ Перейти обратно: а б Чэньгуан, Л.; Женнинг, Ю.; Нефф, Д.; Жаму, А.; Джанг, Б.З. (ноябрь 2010 г.). «Суперконденсатор на основе графена со сверхвысокой плотностью энергии». Нано-буквы . 10 (12): 4863–4868. Бибкод : 2010NanoL..10.4863L . дои : 10.1021/nl102661q . ПМИД 21058713 .
- ^ Миллер-младший; Преступник, РА; Холлоуэй, Британская Колумбия (сентябрь 2010 г.). «Двухслойный графеновый конденсатор с сетевой фильтрацией переменного тока». Наука . 329 (5999): 1637–1639. Бибкод : 2010Sci...329.1637M . дои : 10.1126/science.1194372 . ПМИД 20929845 . S2CID 33772133 .
- ^ Акбулут, С. (2011). Оптимизация электрода суперконденсатора из углеродных нанотрубок (PDF) (магистерская диссертация). Нэшвилл, Теннесси: Аспирантура Университета Вандербильта.
- ^ Перейти обратно: а б Арепалли, С.; Х. Пожарный; К. Хаффман; П. Молони; П. Николаев; Л. Йоуэлл; CD Хиггинс; К. Ким; П. А. Коль; ИП Турано; WJ Ready (2005). «Технологии электрохимических двухслойных конденсаторов на основе углеродных нанотрубок для космических полетов» (PDF) . ДЖОМ . 57 (12): 24–31. Бибкод : 2005JOM....57l..26A . дои : 10.1007/s11837-005-0179-x . S2CID 110891569 . Архивировано из оригинала (PDF) 25 июня 2009 года.
- ^ Перейти обратно: а б Синьорелли, Р.; ДК Ку; Дж.Г. Касакян; Дж. Э. Шиндалл (2009). «Электрохимические двухслойные конденсаторы с использованием электродных структур из углеродных нанотрубок». Учеб. ИИЭЭ . 97 (11): 1837–1847. дои : 10.1109/JPROC.2009.2030240 . hdl : 1721.1/54729 . S2CID 29479545 .
- ^ Ли, Х.; Дж. Ронг; Б. Вэй (2010). «Электрохимическое поведение одностенных суперконденсаторов из углеродных нанотрубок под действием сжимающих напряжений». АСУ Нано . 4 (10): 6039–6049. дои : 10.1021/nn101595y . ПМИД 20828214 .
- ^ Конвей, Бельгия; Бирсс, В. ; Войтович, Дж. (1997). «Роль и использование псевдоемкости для хранения энергии суперконденсаторами». Журнал источников энергии . 66 (1–2): 1–14. Бибкод : 1997JPS....66....1C . дои : 10.1016/S0378-7753(96)02474-3 . hdl : 1880/44956 . S2CID 94810807 .
- ^ Диллон, AC (2010). «Углеродные нанотрубки для фотопреобразования и хранения электрической энергии». хим. Преподобный . 110 (11): 6856–6872. дои : 10.1021/cr9003314 . ПМИД 20839769 .
- ^ Тупен, Матье; Брусс, Тьерри; Беланжер, Даниэль (2004). «Механизм накопления заряда электрода MnO2, используемого в водном электрохимическом конденсаторе». хим. Мэтр . 16 (16): 3184–3190. дои : 10.1021/cm049649j .
- ^ Панг, Сух-Джем; Андерсон, Марк А.; Чепмен, Томас В. (2000). «Новые электродные материалы для тонкопленочных ультраконденсаторов: сравнение электрохимических свойств золь-гель-полученного и электроосажденного диоксида марганца» . Журнал Электрохимического общества . 147 (2): 444–450. Бибкод : 2000JElS..147..444P . дои : 10.1149/1.1393216 .
- ^ Брусс, Тьерри; Беланжер, Даниэль; Лонг, Джеффри В. (1 января 2015 г.). «Быть или не быть псевдоемкостным?» . Журнал Электрохимического общества . 162 (5): А5185–А5189. дои : 10.1149/2.0201505jes . ISSN 0013-4651 .
- ^ Чжэн, Япония (1995). «Гидрооксид рутения как материал электродов для электрохимических конденсаторов». Журнал Электрохимического общества . 142 (8): 2699–2703. Бибкод : 1995JElS..142.2699Z . дои : 10.1149/1.2050077 .
- ^ Дас, Раджиб К.; Лю, Бо; Рейнольдс, Джон Р.; Ринзлер, Эндрю Г. (2009). «Инженерная макропористость в пленках одностенных углеродных нанотрубок». Нано-буквы . 9 (2): 677–683. Бибкод : 2009NanoL...9..677D . дои : 10.1021/nl803168s . ПМИД 19170555 .
- ^ Ван, В.; Го, С.; Ли, И.; Ахмед, К.; Чжун, Дж.; Фаворс, З.; Заера, Ф.; Озкан, М.; Озкан, CS (2014). «Наночастицы водного оксида рутения, прикрепленные к гибридной пене графена и углеродных нанотрубок для суперконденсаторов» . Научные отчеты . 4 : 4452. Бибкод : 2014NatSR...4E4452W . дои : 10.1038/srep04452 . ПМЦ 3964521 . ПМИД 24663242 .
- ^ «Улучшенные суперконденсаторы для более качественных аккумуляторов электромобилей «Библиотека Курцвейла + коллекции» .
- ^ Саймон, Ю.Гогоци (ноябрь 2008 г.). «Материалы для электрохимических конденсаторов» (PDF) . Природные материалы . 7 (11): 845–854. Бибкод : 2008NatMa...7..845S . дои : 10.1038/nmat2297 . ПМИД 18956000 . S2CID 189826716 .
- ^ Конденсатор PAS монетного типа , Тайё Юден, Shoe Electronics Ltd.
- ^ Ли, Синь; Вэй, Бинцин (2012). «Простой синтез и сверхемкостное поведение гибридных пленок SWNT/MnO2». Нано Энергия . 1 (3): 479–487. дои : 10.1016/j.nanoen.2012.02.011 .
- ^ Х. Гуалус и др.: Характеристика и моделирование литий-ионных конденсаторов ESSCAP'08 - 3-й Европейский симпозиум по суперконденсаторам и их применениям, Рим / Италия, 2008 г.
- ^ «FDK начнет массовое производство литий-ионных конденсаторов большой емкости; автомобильная промышленность и возобновляемые источники энергии» . Конгресс зеленых автомобилей. 4 января 2009 года . Проверено 29 мая 2013 г.
- ^ Перейти обратно: а б Наой, Кацухико; Наой, Вако; Аояги, Синтаро; Миямото, Дзюн-Ичи; Камино, Такео (2013). «Новое поколение «Наногибридных суперконденсаторов» ». Отчеты о химических исследованиях . 46 (5): 1075–1083. дои : 10.1021/ar200308h . ПМИД 22433167 .
- ^ Перейти обратно: а б Асайтамби, С.; Сактивел, П.; Каруппайя, М.; Юваккумар, Р.; Баламуруган, К.; Ахамад, Тансир; Хан, М.А. Маджид; Рамалингам, Г.; Мохаммед, Мустафа К.А.; Рави, Г. (1 апреля 2021 г.). «Приготовление нанокомпозитного электрода Fe-SnO2@CeO2 для анализа производительности асимметричных суперконденсаторов» . Журнал хранения энергии . 36 : 102402. doi : 10.1016/j.est.2021.102402 . ISSN 2352-152X . S2CID 233572817 .
- ^ Перейти обратно: а б Мохд Абда, Мухаммад Амирул Айзат; Асман, Нур Хава Набила; Куландайвалу, Шалини; Сулейман, Юсран (14 ноября 2019 г.). «Асимметричный суперконденсатор из функционализированных электроформованных углеродных волокон/поли(3,4-этилендиокситиофена)/оксида марганца//активированного угля с превосходными электрохимическими характеристиками» . Научные отчеты . 9 (1): 16782. Бибкод : 2019НатСР...916782М . doi : 10.1038/s41598-019-53421-w . ISSN 2045-2322 . ПМК 6856085 . ПМИД 31728061 .
- ^ Перейти обратно: а б Хуан, Юаньюань; Ши, Тиелин; Цзян, Шулан; Ченг, Сийи; Тао, Сянсюй; Чжун, Ян; Ляо, Гуанглан; Тан, Цзыжун (7 декабря 2016 г.). «Повышенная циклическая стабильность массивов нанопроволок типа ядро-оболочка NiCo2S4@NiO для полностью твердотельных асимметричных суперконденсаторов» . Научные отчеты . 6 (1): 38620. Бибкод : 2016NatSR...638620H . дои : 10.1038/srep38620 . ISSN 2045-2322 . ПМК 5141571 . ПМИД 27924927 . S2CID 19483393 .
- ^ Перейти обратно: а б Цзян, Цзин; Ли, Чжипенг; Он, Синжуй; Ху, Ялин; Ли, Фу; Хуан, Пей; Ван, Чао (2020). «Новый асимметричный суперконденсатор на основе скуттерудита CoP3 со сверхвысокой плотностью энергии» . Маленький . 16 (31): 2000180. doi : 10.1002/smll.202000180 . ISSN 1613-6829 . ПМИД 32596998 . S2CID 220255613 .
- ^ П. Саймон, А. Берк, Наноструктурный углерод: двухслойная емкость и многое другое.
- ^ Тетрафторборат тетраэтиламмония - Краткое описание соединений CID 2724277 от PubChem
- ^ Перейти обратно: а б Саланн, Матье (30 мая 2017 г.). «Ионные жидкости для применения в суперконденсаторах». Темы современной химии . 375 (3): 63. дои : 10.1007/s41061-017-0150-7 . ISSN 2364-8961 . ПМИД 28560657 . S2CID 22068271 .
- ^ А. Шнойвли, Р. Галлай, Свойства и применение суперконденсаторов, От современного состояния к будущим тенденциям , PCIM 2000
- ^ А. Лафорг и др. Разработка суперконденсаторов нового поколения для транспортных средств. Архивировано 10 января 2014 г. в Wayback Machine.
- ^ Ультраконденсатор Nesscap - Техническое руководство NESSCAP Co., Ltd., 2008 г.
- ^ Перейти обратно: а б с Руководство по продукту Maxwell BOOSTCAP – Ультраконденсаторы Maxwell Technologies BOOSTCAP – Док. № 1014627.1. Архивировано 12 мая 2013 г. в Wayback Machine Maxwell Technologies, Inc., 2009 г.
- ^ Максвелл, серия K2. Архивировано 17 июля 2013 г. в Wayback Machine.
- ^ Перейти обратно: а б с Мартс, Джон (9 мая 2018 г.). Расширенная основанная на физике модель пониженного порядка динамики нефарадеевского электрического двухслойного конденсатора . Цифровые коллекции Колорадо (Диссертация). Университет Колорадо, Колорадо-Спрингс. Семейная библиотека Кремеров. hdl : 10976/166930 .
- ^ (при движении транспортных средств следует учитывать эффективность преобразования энергии, что дает 3700 Втч/кг с учетом типичного КПД двигателя внутреннего сгорания 30%)
- ^ Кристен, Т.; Олер, К. (2002). «Оптимизация компонентов хранения энергии с использованием графиков Рагона». J. Источники питания . 110 (1): 107–116. Бибкод : 2002JPS...110..107C . дои : 10.1016/S0378-7753(02)00228-8 .
- ^ Данн-Рэнкин, Д.; Леал, Э. Мартинс; Вальтер, округ Колумбия (2005). «Личные энергосистемы». Прог. Энергетическое возгорание. Наука . 31 (5–6): 422–465. Бибкод : 2005PECS...31..422D . дои : 10.1016/j.pecs.2005.04.001 .
- ^ Рекомендации по применению Maxwell. Рекомендации по применению — Оценка срока службы модулей накопления энергии. Архивировано 13 июня 2018 г. в Wayback Machine Maxwell Technologies, Inc., 2007 г.
- ^ Panasonic Electronic Devices CO., LTD.: Данные о характеристиках золотых конденсаторов . Архивировано 11 января 2014 г. на Wayback Machine в: Техническое руководство по электрическим двухслойным конденсаторам, издание 7.4, 2011 г.)
- ^ Бонаккорсо, Ф., Коломбо, Л., Ю, Г., Столлер, М., Тоццини, В., Феррари, А., . . . Пеллегрини, В. (2015). Графен, родственные ему двумерные кристаллы и гибридные системы преобразования и хранения энергии. Наука, 1246501-1246501.
- ^ Перейти обратно: а б П. Ван ден Босше и др.: Ячейка против системы: проблемы стандартизации устройств хранения электроэнергии. EVS24 Международный симпозиум по электромобилям, работающим на аккумуляторах, гибридах и топливных элементах, Ставангер/Норвегия, 2009 г.
- ^ Грэм Питчер . Если кепка подойдет .. Архивировано 13 января 2015 года в Wayback Machine . Новая электроника. 26 марта 2006 г.
- ^ «Светодиодный фонарик с ультраконденсатором заряжается за 90 секунд — Slashdot» . Tech.slashdot.org. 10 декабря 2008 года . Проверено 29 мая 2013 г.
- ^ «Гелиевые Bluetooth-колонки с питанием от суперконденсаторов» . Gizmag.com. 27 ноября 2013 года . Проверено 29 ноября 2013 г.
- ^ «Беспроводная отвертка Coleman FlashCell перезаряжается всего за 90 секунд» . О, Гизмо!. 11 сентября 2007 г. Архивировано из оригинала 7 марта 2012 г. Проверено 29 мая 2013 г.
- ^ Перейти обратно: а б М. Фархади и О. Мохаммед, Работа в реальном времени и гармонический анализ изолированных и неизолированных гибридных микросетей постоянного тока , IEEE Trans. Ind. Appl., том 50, № 4, стр. 2900–2909, июль/август. 2014.
- ^ Мангарадж, Мрутюнджая; Панда, Ануп Кумар; Пентия, Трилочан (2016). «Суперконденсатор поддерживает DSTATCOM для снижения гармоник и коррекции коэффициента мощности». Студенческая конференция IEEE по электротехнике, электронике и информатике (SCEECS) 2016 г. стр. 1–6. дои : 10.1109/SCEECS.2016.7509275 . ISBN 978-1-4673-7918-2 . S2CID 16899819 .
- ^ Фархади, Мустафа; Мохаммед, Усама (2015). «Адаптивное управление энергопотреблением в резервированной гибридной микросети постоянного тока для снижения импульсной нагрузки». Транзакции IEEE в Smart Grid . 6 : 54–62. дои : 10.1109/TSG.2014.2347253 . S2CID 37615694 .
- ^ Фархади, Мустафа; Мохаммед, Усама (2015). «Повышение производительности активно управляемой гибридной микросети постоянного тока и импульсной силовой нагрузки». 51 (5): 3570–3578. дои : 10.1109/tia.2015.2420630 . S2CID 17217802 .
{{cite journal}}
: Для цитирования журнала требуется|journal=
( помощь ) - ^ Р. Галлай, Garmanage, технологии и применение суперконденсаторов. Архивировано 30 января 2014 г. в Wayback Machine , Университет Мондрагона, 22 июня 2012 г.
- ^ Дэвид А. Джонсон, ЧП «Суперконденсаторы как накопители энергии» . Откройте для себя сайт Solarenergy.com . Проверено 29 мая 2013 г.
- ^ А. Степанов, И. Галкин, Разработка источника бесперебойного питания на основе суперконденсаторов. Архивировано 11 января 2014 г. в Wayback Machine , Докторантура энергетических и геотехнологий, 15–20 января 2007 г. Курессааре, Эстония.
- ^ «Суперконденсаторный ИБП» . Марафонская сила. Архивировано из оригинала 20 апреля 2013 года . Проверено 29 мая 2013 г.
- ^ Перейти обратно: а б «Maxwell Technologies Ультраконденсаторы (источники бесперебойного питания) Решения для источников бесперебойного питания» . Максвелл.com. Архивировано из оригинала 22 мая 2013 года . Проверено 29 мая 2013 г.
- ^ Международное энергетическое агентство, Программа фотоэлектрических энергетических систем, Роль накопителя энергии для стабилизации мини-сети. Архивировано 14 мая 2013 г. в Wayback Machine , Задача 11 IEA PVPS, Отчет IEA-PVPS T11-02: 2011, июль 2011 г.
- ^ Дж. Р. Миллер, JME, Inc. и Университет Кейс Вестерн Резерв, Конденсаторы для хранения электросетей (Многочасовое массовое хранение энергии с использованием конденсаторов)
- ^ Газанфари, А.; Хамзе, М.; Мохтари, Х.; Карими, Х. (декабрь 2012 г.). «Активное управление питанием мультигибридной системы преобразования энергии топливных элементов / суперконденсаторов в микросети среднего напряжения». Транзакции IEEE в Smart Grid . 3 (4): 1903–1910. дои : 10.1109/TSG.2012.2194169 . ISSN 1949-3053 . S2CID 2107900 .
- ^ Криспо, Рик; Бреккен, Тед К.А. (2013). «Система на основе мотор-генератора и суперконденсатора для стабилизации частоты микросети». 2013 1-я конференция IEEE по технологиям устойчивого развития (Sus Tech ) . стр. 162–166. дои : 10.1109/SusTech.2013.6617314 . ISBN 978-1-4673-4630-6 . S2CID 23894868 .
- ^ Интамуссу, ФА; Пегероль-Керальт, Ж.; Бьянки, Флорида (сентябрь 2013 г.). «Управление суперконденсаторной системой хранения энергии для микросетей». Транзакции IEEE по преобразованию энергии . 28 (3): 690–697. Бибкод : 2013ITEnC..28..690I . дои : 10.1109/TEC.2013.2260752 . hdl : 11336/23530 . ISSN 0885-8969 . S2CID 7454678 .
- ^ Лехтимяки, Суви; Ли, Мяо; Саломаа, Ярно; Пёрхёнен, Юхо; Каланти, Антти; Туукканен, Сампо; Хельо, Петри; Халонен, Кари; Лупо, Дональд (2014). «Производительность печатных суперконденсаторов в схеме сбора радиочастотной энергии». Международный журнал электроэнергетики . 58 : 42–46. Бибкод : 2014IJEPE..58...42L . дои : 10.1016/j.ijepes.2014.01.004 .
- ^ yec.com.tw. «Список поставщиков суперконденсаторов | YEC | Этот высокоэнергетический конденсатор из дефибриллятора может доставить смертельную энергию в 500 джоулей» . ДА. Архивировано из оригинала 11 января 2014 года . Проверено 29 мая 2013 г.
- ^ Перейти обратно: а б «Первый в пути: новый тип запоминающего устройства дает литий-ионным батареям возможность заработать деньги» . Экономист . 12 июля 2014 г.
- ^ Джафар, Амин; Сарени, Бруно; Робоам, Ксавьер; Тиунн-Гермер, Марина (2010). «Размер гибридного локомотива на основе аккумуляторов и ультраконденсаторов». Конференция IEEE по силовым установкам и энергетическим установкам транспортных средств 2010 г. стр. 1–6. дои : 10.1109/VPPC.2010.5729131 . ISBN 978-1-4244-8220-7 . S2CID 26839128 .
- ^ Дж. Р. Миллер, А. Ф. Берк, Электрохимические конденсаторы: проблемы и возможности для реальных приложений , ECS, Vol. 17, № 1, весна 2008 г.
- ^ Fuelcellworks.com. «Дополнительная страница новостей о заводе топливных элементов» . Архивировано из оригинала 21 мая 2008 года . Проверено 29 мая 2013 г.
- ^ ООО «СИНАУТЕК, Автомобильные технологии» . Синаутекус.com. Архивировано из оригинала 8 октября 2013 года . Проверено 29 мая 2013 г.
- ^ М. Фрелих, М. Клор, Санкт-Пагела: Система хранения энергии с UltraCaps на борту железнодорожного транспорта. Архивировано 11 января 2014 г. в Wayback Machine. В: Материалы - 8-й Всемирный конгресс по железнодорожным исследованиям, май 2008 г., Душа, Корея.
- ^ Bombardier, Поддержка энергосбережения MITRAC PDF
- ^ энергосбережения MITRAC в формате PDF Bombardier, Презентация
- ^ «Рейн-Неккар Веркер заказывает больше трамваев с суперконденсаторами» . Железнодорожный вестник . 5 апреля 2011 года. Архивировано из оригинала 8 июня 2011 года . Проверено 29 мая 2013 г.
- ^ «STEEM – содействие энергосбережению для трамваев» . Альстом, СТИМ.
- ^ «Суперконденсаторы будут испытаны в парижском трамвае STEEM» . Железнодорожный вестник . 8 июля 2009 г. Архивировано из оригинала 17 сентября 2011 г. Проверено 29 мая 2013 г.
- ^ «Испытания женевского трамвая оценивают характеристики суперконденсатора» . Железнодорожный вестник . 7 августа 2012 года. Архивировано из оригинала 10 декабря 2012 года . Проверено 29 мая 2013 г.
- ^ «Хранение энергии — глобальный веб-сайт Siemens» . Siemens.com. Архивировано из оригинала 12 мая 2013 года . Проверено 29 мая 2013 г.
- ^ «Суперконденсаторное хранилище энергии для линии Южного острова» . Железнодорожный вестник . 3 августа 2012 года. Архивировано из оригинала 10 декабря 2012 года . Проверено 29 мая 2013 г.
- ^ «Представлен суперконденсаторный легкий поезд метро» . Железнодорожный вестник . 23 августа 2012 года. Архивировано из оригинала 10 декабря 2012 года . Проверено 29 мая 2013 г.
- ^ Перейти обратно: а б с д «Первый по дороге» . Экономист . 10 июля 2014 г.
- ^ Первый суперконденсаторный трамвай со 100% низким полом в Ухане начинает первый пробный запуск , China News Network, 31 мая 2016 г.
- ^ «4-Neo Green Power» (PDF) . Архивировано из оригинала (PDF) 10 января 2014 года . Проверено 23 октября 2013 г.
- ^ «UITP 2015: Alstom запускает SRS, новую наземную систему статической зарядки, и расширяет свое решение APS для автомобильного транспорта» . www.alstom.com . Проверено 4 ноября 2017 г.
- ^ «Интегрированная трамвайная система Alstom начинает коммерческую эксплуатацию в Рио за несколько месяцев до Олимпийских игр» . www.alstom.com . Проверено 4 ноября 2017 г.
- ^ «Ultracapbus - VAG Нюрнберг - Местный общественный транспорт в Нюрнберге» . Ваг.де. Архивировано из оригинала 11 января 2014 года . Проверено 29 мая 2013 г.
- ↑ Стефан Кершль, Эберхард Хипп, Джеральд Лексен: Эффективный гибридный привод с ультракапами для городских автобусов. Архивировано 11 января 2014 г. на Wayback Machine , 2005 г. (немецкий). 14-м Ахенском коллоквиуме
- ^ В. Харри, С. Эйген, Б. Земп, Д. Карьеро: Микроавтобус «TOHYCO-Rider» с хранилищем сверхемкости SAM. Архивировано 11 января 2014 г. в Годовой отчет Wayback Machine за 2003 г. - программа «Трафик и аккумуляторы», HTA Люцерн. , Университет прикладных наук Центральной Швейцарии (Германия)
- ^ Гамильтон, Тайлер (19 октября 2009 г.). «Следующая остановка: ультраконденсаторные шины | Обзор технологий MIT» . Technologyreview.com. Архивировано из оригинала 26 марта 2013 года . Проверено 29 мая 2013 г.
- ^ «Представлен зеленый микроавтобус «трибрид»» . Би-би-си . 5 июня 2008 года . Проверено 12 января 2013 г.
- ^ «Запуск первого в Европе трибридного зеленого микроавтобуса» . 30 мая 2008 г. Архивировано из оригинала 11 января 2014 г. . Проверено 12 января 2013 г.
- ^ Формула-1 2011: Система регулирования силовых агрегатов. Архивировано 17 февраля 2012 года в Wayback Machine . 24 мая 2007 г. Проверено 23 апреля 2013 г.
- ^ «Большой анализ: KERS для чайников – Формула-1» . Motorsport-total.com. 25 мая 2013 года . Проверено 29 мая 2013 г.
- ^ «Представлен гибрид Toyota TS030 LMP1» . Машиностроение гоночных автомобилей . 24 января 2012 года . Проверено 30 мая 2013 г.
- ^ Шуриг, Маркус (9 апреля 2012 г.). «Гибридная технология в Toyota TS030: суперконденсаторы к успеху в Ле-Мане» .
- ^ Фред Жайе (15 июня 2012 г.). «Пост TOYOTA Racing впечатляет в квалификации Ле-Мана • TOYOTA Racing — команда чемпионата мира по гонкам на выносливость FIA» . Toyotahybridracing.com . Проверено 30 мая 2013 г.
- ^ А. Ф. Берк, Аккумуляторы и ультраконденсаторы для электромобилей, гибридов и транспортных средств на топливных элементах. Архивировано 7 января 2014 г. в Wayback Machine.
- ^ Суперконденсаторы Cap-XX для автомобилей и других транспортных средств. Архивировано 19 июня 2013 г. в Wayback Machine , март 2012 г.
- ^ А. Песаран, Дж. Гондер, Недавний анализ UCAP в мягких гибридах. Архивировано 7 октября 2012 г. в Wayback Machine , Национальная лаборатория возобновляемых источников энергии, Голден, Колорадо, 6-я конференция по передовым автомобильным батареям, Балтимор, Мэриленд, 17–19 мая 2006 г.
- ^ AFS TRINITY ПРЕДСТАВЛЯЕТ Внедорожник EXTREME HYBRID (XH™) с расходом топлива 150 миль на галлон. Архивировано 29 февраля 2012 года в Wayback Machine . AFS Trinity Power Corporation. 13 января 2008 г. Проверено 31 марта 2013 г.
- ^ Росс, Джеффри Н. «Mazda6 i-Eloop 2014 года с расходом топлива 40 миль на галлон по шоссе и 28 миль на галлон в городе» .
- ^ А.Е. КРАМЕР, миллиардер поддерживает создание газоэлектрического гибридного автомобиля в России, The New York Times, 13 декабря 2010 г. [2]
- ^ Лондонская авиакомпания Emirates Air Line: самая дорогая канатная дорога в мире с сомнительной значимостью для трафика.
- ^ ISR, Internationale Seilbahn Rundschau, Лучшее развлечение над облаками
- ^ Ян, X.; Ченг, К.; Ван, Ю.; Ли, Д. (август 2013 г.). «Плотная интеграция графеновых материалов с помощью жидкости для компактного емкостного хранения энергии». Наука . 341 (6145): 534–537. Бибкод : 2013Sci...341..534Y . дои : 10.1126/science.1239089 . ПМИД 23908233 . S2CID 206549319 .
- ^ Фасткап. «Смена парадигмы» . Системы ФастКап. Архивировано из оригинала 21 июня 2013 года . Проверено 30 мая 2013 г.
- ^ «Новый углеродный материал улучшает суперконденсаторы» . Rsc.org. 13 мая 2011 года . Проверено 1 марта 2015 г.
- ^ Ю. Чжу; и др. (май 2011 г.). «Углеродные суперконденсаторы, полученные путем активации графена». Наука . 332 (3067): 1537–1541. Бибкод : 2011Sci...332.1537Z . дои : 10.1126/science.1200770 . ПМИД 21566159 . S2CID 10398110 .
- ^ Ким, Тайвань; Юнг, Г.; Ю, С.; Эх, КС; Руофф, РС (июль 2013 г.). «Активированный уголь на основе графена как электроды суперконденсатора с макро- и мезопорами». АСУ Нано . 7 (8): 6899–6905. дои : 10.1021/nn402077v . ПМИД 23829569 . S2CID 5063753 .
- ^ «Микропористый полимерный материал для суперконденсаторов с большой емкостью, высокой плотностью энергии и мощности и превосходным сроком службы» . Конгресс зеленых автомобилей.
- ^ Коу, Ян; Сюй, Яньхун; Го, Чжаоци; Цзян, Дунлинь (2011). «Сверхемкостное хранение энергии и электроснабжение с использованием аза-сопряженного π-микропористого каркаса». Ангеванде Хеми . 50 (37): 8753–8757. Бибкод : 2011АнгЧ.123.8912К . дои : 10.1002/ange.201103493 . ПМИД 21842523 .
- ^ Изади-Наджафабади, А.; Ямада, Т.; Футаба, ДН; Юдасака, М.; Такаги, Х.; Хатори, Х.; Иидзима, С.; Хата, К. (2011). «Мощные суперконденсаторные электроды из одностенного композита углеродных нанорогов и нанотрубок» . АСУ Нано . 5 (2): 811–819. дои : 10.1021/nn1017457 . ПМИД 21210712 .
- ^ Тан, Чжэ; Чунь-хуа, Тан; Гонг, Хао (2012). «Асимметричный суперконденсатор с высокой плотностью энергии из наноструктурированных электродов Ni(OH)2/углеродных нанотрубок» . Адв. Функц. Мэтр . 22 (6): 1272–1278. дои : 10.1002/adfm.201102796 . S2CID 93356811 .
- ^ Чиен, Син-Чи; Ченг, Вэй-Юнь; Ван, Юн-Хуэй; Лу, Ши-Юань (2012). «Сверхвысокие удельные емкости суперконденсаторов, достигаемые с помощью композитов никель-кобальтит/углеродный аэрогель». Передовые функциональные материалы . 22 (23): 5038–5043. дои : 10.1002/adfm.201201176 . S2CID 97810530 .
- ^ Почта; Ли, Х; Чжао, Ю; Сюй, Л; Сюй, Х; Ло, Ю; Чжан, З; Ке, Вт; Ню, К; Чжан, К. (2013). «Наночешуйчатый электрод с возможностью быстрой ионной диффузии путем спонтанной электрохимической предварительной интеркаляции для высокопроизводительных суперконденсаторов» . Научный представитель . 3 : 1718. Бибкод : 2013NatSR...3E1718M . дои : 10.1038/srep01718 . ПМК 3634106 .
- ^ Занг, Л.; и др. (2014). «Пористые объемные материалы на основе трехмерного графена с исключительно большой площадью поверхности и превосходной проводимостью для суперконденсаторов» . Научный представитель . 3 : 1408. Бибкод : 2013NatSR...3E1408Z . дои : 10.1038/srep01408 . ПМК 3593215 . ПМИД 23474952 .
- ^ У, Чжун-Шуай; Фэн, Синьлян; Ченг, Хуэй-Мин (2013). «Последние достижения в области планарных микросуперконденсаторов на основе графена для хранения энергии на кристалле» . Национальный научный обзор . 1 (2): 277–292. дои : 10.1093/nsr/nwt003 .
- ^ «Сверхтонкие конденсаторы могут ускорить разработку электроники следующего поколения | KurzweilAI» . www.kurzweilai.net . 28 февраля 2016 г. Проверено 11 февраля 2014 г.
- ^ Ван, Чэнсян; Осада, Минору; Эбина, Ясуо; Ли, Бао-Вэнь; Акацука, Кошо; Фукуда, Кацутоши; Сугимото, Ватару; Ма, Ренжи; Сасаки, Такаёси (19 февраля 2014 г.). «Полностью нанолистовые ультратонкие конденсаторы, собранные слой за слоем с помощью процессов, основанных на решениях». АСУ Нано . 8 (3): 2658–2666. дои : 10.1021/nn406367p . ПМИД 24548057 . S2CID 7232811 .
- ^ Боргино, Дарио (19 апреля 2015 г.). «Новое устройство сочетает в себе преимущества аккумуляторов и суперконденсаторов» . www.gizmag.com . Проверено 10 февраля 2016 г.
- ^ «Гибкие 3D-графеновые суперконденсаторы могут использоваться в портативных и носимых устройствах | KurzweilAI» . www.kurzweilai.net . Проверено 11 февраля 2016 г. .
- ^ Пэн, Живэй; Линь, Цзянь; Йе, Жуцюань; Сэмюэл, Эррол Л.Г.; Тур, Джеймс М. (28 января 2015 г.). «Гибкие и штабелируемые графеновые суперконденсаторы, индуцированные лазером». Прикладные материалы и интерфейсы ACS . 7 (5): 3414–3419. дои : 10.1021/am509065d . ПМИД 25584857 .
- ^ "Прорыв батареи заряжается за секунды, хватает на неделю | KurzweilAI" . www.kurzweilai.net . 25 ноября 2016 года . Проверено 2 февраля 2017 г.
- ^ Чоудхари, Нитин; Ли, Чао; Чунг, Хи-Сук; Мур, Джулиан; Томас, Джаян; Юнг, Ёнун (27 декабря 2016 г.). «Высокопроизводительный однокорпусный нанопроволочный суперконденсатор с одним корпусом, реализованный за счет конформного роста емкостных 2D-слоев WS2». АСУ Нано . 10 (12): 10726–10735. дои : 10.1021/acsnano.6b06111 . ISSN 1936-0851 . ПМИД 27732778 .
- ^ Раут, А.; Паркер, К.; Гласс, Дж. (2010). «Метод получения графика Рагона для оценки электродов суперконденсатора из углеродных нанотрубок». Журнал исследования материалов . 25 (8): 1500–1506. Бибкод : 2010JMatR..25.1500R . дои : 10.1557/JMR.2010.0192 . S2CID 110695012 .
- ^ «В 2016 году мировой рынок суперконденсаторов столкнется с уникальными проблемами» . РынокГЛАЗ. 10 марта 2016 г. Архивировано из оригинала 3 ноября 2016 г. . Проверено 19 марта 2017 г.
- ^ Деннис Зогби, Paumanok Group, 4 марта 2013 г., Суперконденсаторы - миф, потенциал и реальность.
- ^ «Суперконденсаторные технологии и рынки 2016-2026» . ИДТехЭкс. 1 ноября 2016 года . Проверено 10 марта 2017 г.
- ^ Обзор рынка T2+2™. Архивировано 16 мая 2011 г. в Wayback Machine , гл. Ахерн, Суперконденсаторы, 10 декабря 2009 г., номер проекта NET0007IO.
Дальнейшее чтение
[ редактировать ]- Татрари, Г.; Ахмед, М.; Шах, ФУ (2024). «Синтез, термоэлектрические и энергоаккумулирующие свойства композитов на основе оксидов переходных металлов». Обзоры координационной химии . 498 : 215470. doi : 10.1016/j.ccr.2023.215470 .
- Абрунья, HD; Кия, Ю.; Хендерсон, Дж. К. (2008). «Батареи и электрохимические конденсаторы» (PDF) . Физ. Сегодня . 61 (12): 43–47. Бибкод : 2008ФТ....61л..43А . дои : 10.1063/1.3047681 . Архивировано из оригинала (PDF) 4 марта 2016 года . Проверено 17 февраля 2015 г.
- Бокрис, Дж. О'М; Деванатан, МАВ; Мюллер, К. (1963). «О структуре заряженных интерфейсов». Учеб. Р. Сок. А. 274 (1356): 55–79. Бибкод : 1963РСПСА.274...55Б . дои : 10.1098/rspa.1963.0114 . S2CID 94958336 .
- Беген, Франсуа; Раймундо-Пиньейро, Э.; Фраковяк, Эльжбета (2009). «Электрические двухслойные конденсаторы и псевдоконденсаторы». Углероды для электрохимических систем хранения и преобразования энергии . Передовые материалы и технологии. Том. 20091238. CRC Press. стр. 329–375. дои : 10.1201/9781420055405-c8 . ISBN 978-1-4200-5540-5 .
- Конвей, Брайан Эванс (1999). Электрохимические суперконденсаторы: научные основы и технологические приложения . Спрингер . дои : 10.1007/978-1-4757-3058-6 . ISBN 978-0306457364 .
- Чжан, Дж.; Чжан, Л.; Лю, Х.; Солнце, А.; Лю, Р.-С. (2011). «8. Электрохимические суперконденсаторы» . Электрохимические технологии хранения и преобразования энергии . Вайнхайм: Wiley-VCH. стр. 317–382. ISBN 978-3-527-32869-7 .
- Лейтнер, К.В.; Зима, М.; Безенхард, Дж.О. (2003). «Композитные электроды суперконденсатора». J. Твердотельные электрики . 8 (1): 15–16. дои : 10.1007/s10008-003-0412-x . S2CID 95416761 .
- Киносита, К. (18 января 1988 г.). Углерод: электрохимические и физико-химические свойства . Джон Уайли и сыновья. ISBN 978-0-471-84802-8 .
- Вольфкович Ю.М.; Сердюк, ТМ (2002). «Электрохимические конденсаторы». Расс. Дж. Электрохим . 38 (9): 935–959. дои : 10.1023/А:1020220425954 .
- Паланисельвам, Тангавелу; Пэк, Чон Бом (2015). «2D-материалы на основе графена для суперконденсаторов». 2D материалы . 2 (3): 032002. Бибкод : 2015TDM.....2c2002P . дои : 10.1088/2053-1583/2/3/032002 . S2CID 135679359 .
- Плен, Гарри (2015). «Композит для хранения энергии забирает тепло» . Природа . 523 (7562): 536–537. Бибкод : 2015Natur.523..536P . дои : 10.1038/523536а . ПМИД 26223620 . S2CID 4398225 .
- Ли, Цюй (2015). «Гибкие высокотемпературные диэлектрические материалы из полимерных нанокомпозитов». Природа . 523 (7562): 576–579. Бибкод : 2015Natur.523..576L . дои : 10.1038/nature14647 . ПМИД 26223625 . S2CID 4472947 .